Back to Journals » International Journal of Nanomedicine » Volume 19
Applications of Graphene Family Nanomaterials in Regenerative Medicine: Recent Advances, Challenges, and Future Perspectives
Authors Chen X, Zou M, Liu S, Cheng W, Guo W, Feng X
Received 13 February 2024
Accepted for publication 14 May 2024
Published 7 June 2024 Volume 2024:19 Pages 5459—5478
DOI https://doi.org/10.2147/IJN.S464025
Checked for plagiarism Yes
Review by Single anonymous peer review
Peer reviewer comments 2
Editor who approved publication: Dr Kamakhya Misra
Xiuwen Chen,1,* Meiyan Zou,1,* Siquan Liu,1 Weilin Cheng,1 Weihong Guo,2 Xiaoli Feng1
1Stomatology Hospital, School of Stomatology, Southern Medical University, Guangzhou, People’s Republic of China; 2Department of General Surgery, Nanfang Hospital, The First School of Clinical Medicine, Southern Medical University, Guangzhou, People’s Republic of China
*These authors contributed equally to this work
Correspondence: Weihong Guo; Xiaoli Feng, Email [email protected]; [email protected]
Abstract: Graphene family nanomaterials (GFNs) have attracted considerable attention in diverse fields from engineering and electronics to biomedical applications because of their distinctive physicochemical properties such as large specific surface area, high mechanical strength, and favorable hydrophilic nature. Moreover, GFNs have demonstrated the ability to create an anti-inflammatory environment and exhibit antibacterial effects. Consequently, these materials hold immense potential in facilitating cell adhesion, proliferation, and differentiation, further promoting the repair and regeneration of various tissues, including bone, nerve, oral, myocardial, and vascular tissues. Note that challenges still persist in current applications, including concerns regarding biosecurity risks, inadequate adhesion performance, and unsuitable degradability as matrix materials. This review provides a comprehensive overview of current advancements in the utilization of GFNs in regenerative medicine, as well as their molecular mechanism and signaling targets in facilitating tissue repair and regeneration. Future research prospects for GFNs, such as potential in promoting ocular tissue regeneration, are also discussed in details. We hope to offer a valuable reference for the clinical application of GFNs in the treatment of bone defects, nerve damage, periodontitis, and atherosclerosis.
Keywords: graphene, graphene oxide, regenerative medicine, scaffold, anti-inflammatory, bone regeneration, nerve, angiogenesis, dental pulp stem cells
Graphical Abstract:
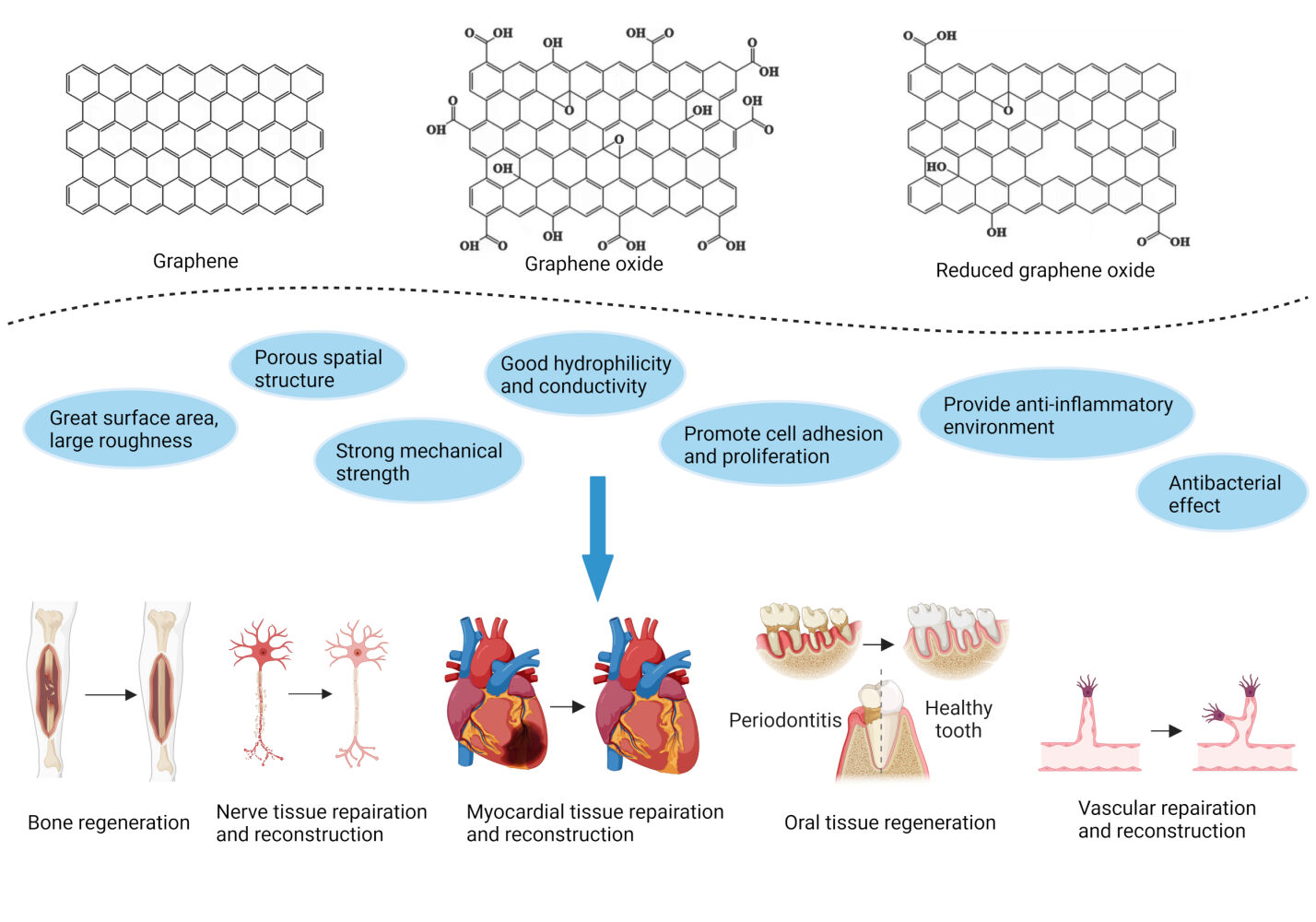
Introduction
Graphene is a carbon-based nanomaterial comprising carbon atoms that are linked by sp² hybridization and are tightly packed into a single-layer, two-dimensional (2D) honeycomb lattice structure. Its chemical and physical modifications can form various graphene-related materials, including monolayer or multilayer graphene, graphene oxide (GO), reduced graphene oxide (rGO), etc. These graphene family nanomaterials (GFNs) possess high mechanical strength, strong chemical stability, excellent thermal and electrical conductivity, and unique optical behavior.1 More importantly, growing evidence has shown that GFNs exhibit tremendous application potential in biomedical fields because of their adjustable property and active biological behavior. For example, GO-Polyethylene glycol (PEG) 6000 is made by bridging PEG with GO, which has been used to load fluorescein (Flu) as a fluorescent probe for cell imaging.2 Additionally, polyamide-amine-modified GO was reported to deliver the MMP-9 shRNA gene drug to induce apoptosis of MCF-7 cells (human breast cancer cells), or to deliver cyclosporin A to reduce the rejection and excretion of MCF-7 cells towards chemotherapy drug (doxorubicin), thereby enhancing anti-tumor effects.3 Furthermore, novel nanomaterials made of graphene and zinc oxide coated on the surface of plastic teeth or oral implant can inhibit the formation of Streptococcus mutans biofilm.4
Regenerative medicine is a new discipline and frontier interdisciplinary field that applies biological and engineering methods to repair, rebuild, regenerate, and improve aberrant tissue and organ functions. In recent years, the applications of GFNs in regenerative medicine have attracted much attention. Traditional scaffold materials used in tissue engineering possess good biocompatibility and hydrophilicity, but low mechanical strength and poor degradation controllability limit their applications. Adding rGO and GO with suitable diameter and appropriate concentration can improve the mechanical strength of the composite scaffold material, make it amphiphilic, and improve its biological activity.5,6 Moreover, rGO can participate in the formation of the sustained drug release system, which upregulates the mRNA expression of osteogenic markers like ALP, BMP-2, and RUNX-2 by loading and releasing Teriparatide, thus enhancing bone regeneration.7 Other reported applications of GFNs in regenerative medicine also contain hydrogels, nanofibers, and conductive composite films, which act on angiogenesis,8 and the repair and reconstruction of nerve tissue9 and myocardial tissue,10 respectively.
To further explore the mechanism of action of GFNs in regenerative medicine, it mainly includes adsorbing proteins to increase cell adhesion and proliferation, regulating the expression of key genes and proteins, providing an anti-inflammatory environment, exerting an antibacterial effect, connecting to extracorporeal electrical stimulation, etc.11–14 To be more specific, GO has been combined with the gelatin sponge (GS) to construct composite biological scaffolds, which are loaded with hDPSCs via LMP-1 transfection for being used in dentin and bone tissue engineering.15 Additionally, the bilayer neural catheters drug loading system containing Gelatin/Chitosan(CS)/Poly-DL-lactide(PDLLA)/GO/Vascular endothelial growth factor (VEGF) is developed to be used for neural tissue repair. Its inner layer is loaded with drug factors, and the outer layer possesses electrical conductivity derived from GO.16 However, it is worth noting that there are still some problems in their current applications, such as biosecurity risks,17 insufficient bonding strength between graphene and titanium implant,18 and inappropriate degradability as a matrix material.19 Despite these limitations, the investigation into the biological implications of GFNs continues to expand and advance. Recent evidence has demonstrated the capability of GO and rGO to facilitate the regeneration of ocular tissue through fostering the adhesion, growth, and proliferation of diverse human ocular cell lines and primary cells.20,21 Nevertheless, there is limited research in this area, and more ongoing and comprehensive research efforts are still required in the future.
This review summarizes the research progress of the GFNs in the repair and reconstruction of various tissues based on their physicochemical properties and unique biological behaviors. The related cellular and molecular mechanisms are also comprehensively discussed. Finally, current challenges of GFNs’ applications and new prospects for their future medical potentials are suggested. This article hopes to provide abundant experimental references and new therapeutic ideas for GFNs’ clinical applications and further research prospects in regenerative medicine.
Physicochemical Properties of the GFNs
Surface Area and Roughness
The specific surface area of GFNs exceeds 2000m2/g, surpassing the surface area of most other nanomaterials by more than tenfold. The surfaces and edges of these nanomaterials possess the capability to interact with biomolecules. An example of this interaction is observed when GO is combined with deacetyl-chitosan (CS), resulting in the formation of a CS-GO scaffold characterized by enhanced roughness and surface area. Consequently, a greater number of human bone marrow mesenchymal stem cells (hMSCs) adhered to the composite scaffold, leading to superior regeneration of the bone tissue.22
Porous Spatial Structure
The osteogenic differentiation of stem cells and bone regeneration can be facilitated by the porous structure of graphene-related nanomaterials.23 Previous research has indicated that the accumulation of π-π bonds and van der Waals forces between two-dimensional (2D) graphene sheets results in the formation of a thin layer on the surface of 3D graphene scaffolds, which possess a porous spatial structure.24 This porous structure enables the penetration and diffusion of nutrients, facilitating cell attachment, proliferation, and creating favorable conditions for cellular growth and development.
Mechanical Strength
Graphene exhibits a robust atomic structure wherein each carbon atom forms three robust σ bonds with neighboring carbon atoms. Consequently, GFNs surpass diamond in strength, rendering it the epitome of material strength. By incorporating GO into established scaffold materials like chitosan, enhancements in mechanical properties, encompassing compressive and flexural strength, can all be achieved.25
Hydrophilicity
The ionization of the hydroxyl and carboxyl groups of GO in aqueous solution leads to the dispersion of hydrogen ions, resulting in a negatively charged GO surface. The repulsive force between negative charges effectively prevents the agglomeration of GO in water, thereby facilitating its stable dispersion.26 Moreover, the favorable hydrophilicity of GO enables an enhanced concentration of carrier drugs and promotes their biological activity.
Conductivity
Graphene exhibits exceptional conductivity, with its electrons demonstrating a conduction rate at room temperature that is 1/300 (106m/s) of the speed of light. This rate surpasses that of conventional semiconductors like germanium and silicon.27 Leveraging this property, GFNs can be employed for the conduction of exogenous electrical stimulation, thereby facilitating tissue cell proliferation and differentiation. Currently, they have been served as outstanding scaffold materials for neural28,29 and myocardial30,31 tissue engineering.
Biological Performance of GFNs in Regenerative Medicine
Promote Cell Adhesion and Proliferation
The previous studies have recognized that the adhesion of cells to materials is affected by the surface area and hydrophilicity of the materials.32 For instance, low-oxygen graphene (LOG) demonstrates a significant specific surface area and favorable hydrophilicity, allowing it to efficiently adsorb adhesion proteins like fibronectin and vitronectin, thereby promoting cell adhesion and proliferation.33 Additionally, the structural characteristics of graphene foams can effectively enhance the development of focal adhesions in neural stem cells (NSCs) by providing a greater number of focal adhesion points.34
Provide an Anti-Inflammatory Environment
The modulation of immune responses from a pro-inflammatory state to an anti-inflammatory state following the introduction of biomaterials is regarded as an additional crucial step in facilitating proper tissue healing.35 When exposed to different environmental stimulation, human macrophages differentiate into specific phenotypes, including classical activated macrophage (M1) and alternatively activated macrophage (M2) polarization, and make specific functional reactions. M1 macrophages up-regulate the expression of pro-inflammatory cytokines, such as tumor necrosis factor-alpha (TNF-α), interleukin-1α (IL-1α), IL-1β, IL-6 and produces reactive oxygen species (ROS) to induce tissue damage. In comparison, macrophage M2 polarization stimulates the release of anti-inflammatory cytokines like IL-10 and transforming growth factor beta (TGF-β).36 It has been established that GO possesses the ability to induce the secretion of anti-inflammatory cytokines in macrophages by promoting the transformation of M1 into M2 macrophages.37 Furthermore, the Toll-like receptor 4-dependent expression of IL-1β and IL-6 mRNA can be attenuated following GO exposure, thereby exerting inhibitory effects on the inflammatory response.38 Through these mechanisms, GO creates a microenvironment that is conducive to osteogenesis and angiogenesis, both of which are integral to the process of bone regeneration.23 Similarly, based on its conductivity, rGO has the potential to enhance the restoration of volumetric muscle loss by mitigating the inflammatory response and stimulating vascularization, manifested as downregulation of IL-6 and upregulation of VEGF.39
Antibacterial Function
Akhavan et al40 demonstrated that the presence of numerous polymerized GO sheets in the suspension effectively encapsulated bacteria, thereby isolating them from the external environment and impeding the absorption of essential nutrients, finally inhibiting their growth. The author further expanded on this finding by suggesting that the sharp edges of GO also exhibited bactericidal properties through the physical severing of the bacterial cell membrane.41 Furthermore, GO was observed to extract phospholipids from the cell membrane, leading to the disruption of the bilayer structure, and this effect is size-dependent.42 Previous studies have provided evidence of the ability of GFNs to hinder bacterial growth by inducing oxidative stress through two potential mechanisms: 1) by causing an imbalance in intracellular redox status through generating ROS; 2) by disrupting bacterial metabolic processes via oxidizing intracellular structures or components. For example, Wang et al43 discovered that the exposure of graphene led to the inhibition of bacterial activity in Porphyromonas gingivalis (P. gingivalis) and Candida albicans (C. albicans) by inducing ROS production. Similarly, GO and rGO-induced glutathione oxidation in Escherichia coli (E. coli) also resulted in bacteriostatic action, which was positively correlated with the dose and duration of nanomaterial exposure.
In addition to inherent antibacterial properties, GO possesses a high concentration of oxygen-containing active groups on its surface, enabling it to effectively bind with antibacterial drugs via chemical binding, further enhancing the antibacterial effect. The loading of Zn2+ onto GO facilitated the binding with thiol-containing proteins and enzymes present on the surface of Streptococcus mutans, leading to membrane rupture.11,44 GO-loaded silver nanoparticles also disrupted the membrane integrity of E. coli and Staphylococcus aureus (S. aureus), resulting in increased membrane permeability. Ag+ released from AgNPs additionally contributed to bacterial eradication by inducing oxidative stress.45,46 The introduction of rGO has also been observed to facilitate drug loading, thereby enhancing antibacterial activity. It was revealed that the rGO coating on the titanium plate effectively impeded the growth of both S. aureus and E. coli by inducing membrane stress and generating ROS. Furthermore, the rGO coating exhibited an enhanced capacity for levofloxacin loading and release, exerting a synergistic antibacterial effect.47
Role in Biological Scaffolds
The utilization of GFNs presents an opportunity to enhance the mechanical strength and protein adsorption capacity of composite biological scaffolds, thus facilitating cell adhesion and proliferation. In a study conducted by Wen,48 the graphene membrane was successfully applied as a coating on the chitosan matrix for improving its compressive strength. Subsequently, a greater number of L929 cells (mouse fibroblasts) exhibited close adherence to the scaffold’s surface with improved cellular morphology. The utilization of GO nanoparticles also presents a potential avenue for enhancing the mechanical strength and hydrophilicity of PLGA scaffolds, which effectively facilitated the adhesion and proliferation of MC3T3-E1 cells (mouse embryonic osteoblast precursor cells). These composites additionally stimulate cell differentiation by activating the FAK/P38 signaling pathway and up-regulating osteogenic differentiation genes like OCN and Rux2.49 Similarly, the incorporation of rGO within the hydroxyapatite scaffold imparts a uniform porous structure and heightened mechanical strength, consequently augmenting cell adhesion, proliferation, and spontaneous osteogenic differentiation.50
Role in Semi-Fluid Hydrogels
Hydrogels, comprised of hydrophilic macromolecules or polymers, exhibit a substantial water content and possess the capacity to replicate the 3D microenvironment of the extracellular matrix. As a result, hydrogels present significant prospects in the field of biomedical engineering. The incorporation of graphene and/or its derivatives into hydrogels can additionally augment the mechanical robustness, self-repairing abilities, and light responsiveness of these substances. Zhang et al introduced 0.8% (mass ratio) of GO into polyvinyl alcohol (PVA) hydrogel, thus increased its tensile and compressive strength by 132% and 36%, respectively, compared to the raw material.51 It has been observed that polyacrylamide (PAM) hydrogel tends to swell and fracture in deionized water, but the GO/PAM composite hydrogel exhibits superior self-repair capability, as it maintains its original state.52 Moreover, the incorporation of graphene into PNIPAM enhances its porous structure, resulting in an increased equilibrium swelling rate and accelerated de-swelling rate. Consequently, the temperature sensitivity and delivery rate of heat-targeted drugs are improved.53 The PNIPAM-GO composites also demonstrate a notable alteration in volume upon near-infrared light irradiation due to the adsorption of these radiations by GO. The temperature rise and the volume contraction rate are both accelerated with higher GO content, and this effect is reversible and repeatable. For these reasons, the PNIPAM-GO composite hydrogel is expected to have significant implications in addressing bone defects in various anatomical sites, including skull defects and maxillofacial bone defects.54
Applications of the GFNs in Regenerative Medicine
Bone Regeneration
It has been evidenced that GO can enhance the osteogenic potential of stem cells. Scaffolds containing GO can promote the proliferation and differentiation of human bone marrow mesenchymal stem cells (hBMSCs) through increasing the expression of osteogenic genes and enhancing ALP activity.55 Tabatabaee et al also indicated that poly-2-hydroxyethyl methacrylate-gelatin-GO scaffold could effectively mediate hBM-MSCs differentiation into osteoblasts without the addition of osteogenic differentiation medium.56 The scaffold’s swelling is an important property, as during swelling, the scaffold’s porosity and pore size increase, which promotes cell adhesion, proliferation, and migration, and then helps bone formation. GO addition can increase the swelling degree of the silver-coated polymer nanocomposite scaffolds.57 Additionally, it has been found that GFNs induce osteogenesis by modulating key signaling pathways. For instance, graphene exerts osteoinductive effects by activating the PI3K/Akt/GSK-3β/β-catenin signaling pathway; rGO in ZnHA-rGO nanosystem induces actin polymerization by stimulating integrin, thus increasing Ca2+ entry into the cell that activates osteogenesis-related Hippo-Yes-associated protein (YAP) and TAZ transcription factors, ultimately promoting osteogenesis.58 In the in vivo study, GO incorporation into silk fibroin/nano-hydroxyapatite scaffolds can inhibit M1-like macrophage differentiation to reduce the early inflammatory response in the skull defect of rats and promote the M2-like macrophage differentiation in the middle and late inflammatory stages to accelerate tissue repair.13 Similarly, PGO (polydopamine-reduced graphene oxide) could endow the PHA (hydroxyapatite nanoparticle)-AG (alginate/gelatin) scaffold with good electrical conductivity and up-regulate osteogenic gene expressions of BMSCs under high throughput electrical stimulation (ES). In the meantime, ROS was cleared and HIF-1 signaling pathway down-regulated, thereby inhibiting the polarization of M1 macrophages and the secretion of pro-inflammatory factors, which is conducive to bone formation. Four weeks after the scaffold was implanted into the type I diabetic rat mandibular periodontal fenestration model, the new bone formed in the defect area was larger in volume and higher in density (demonstrated in Figure 1).59 This suggests that regulating the immune microenvironment may be a breakthrough point in promoting periodontal bone regeneration in diabetes patients.
![]() |
Figure 1 Cell adhesion and high throughput electrical stimulation (ES) of BMSCs on the surfaces of scaffolds. (a) Cell morphologies of BMSCs on the surfaces of AG, PHA-AG, and PGO-PHA-AG scaffolds (10 wt ‰ PGO). (b) Cell spreading area of BMSCs. (c) Proliferation of BMSCs on the various scaffolds after 3 and 7 d of culturing. (d) Schematic of the high throughput ES. The conductive scaffold delivered electrical signals to BMSCs, which resulted in a Ca2+ influx. Then, intracellular signaling cascades were activated, which contributed to the up-regulation of genes related to osteogenesis and eventually promoted the osteogenesis of BMSCs. (e) Alkaline phosphatase (ALP) activity of BMSCs on AG, PHA-AG, and PGO-PHA-AG scaffolds (10 wt ‰ PGO) under various ES potentials after 14 d. (f–h) Osteogenesis-related gene expressions of collagen type I alpha 1 (COL1A1), Osterix (Osx), and ALP with or without ES after 14 d. (i) Flow cytometry of Fluo-4 AM-labeled cells in the fluorescein isothiocyanate (FITC)-A channel, indicating intracellular Ca2+ concentration of cells on the PGO-PHA-AG scaffold (10 wt ‰ PGO) with or without ES. The data are expressed as mean ± standard deviation with n≥3. *P < 0.05 and **P < 0.01 were considered statistically significant. Notes: Reproduced from Y. Li, L. Yang, Y. Hou, Z. Zhang, M. Chen, M. Wang, J. Liu, J. Wang, Z. Zhao, C. Xie, X. Lu. Polydopamine-mediated graphene oxide and nanohydroxyapatite- incorporated conductive scaffold with an immunomodulatory ability accelerates periodontal bone regeneration in diabetes, Bioact Mater 18 (2022) 213–227. Creative Commons.59 |
Nerve Tissue Repair and Reconstruction
GFNs can be used to promote the repair and regeneration of nerve tissue because ①they have good conductivity so as to promote intercellular signal transduction; ②they can enhance the mechanical strength of the stent and support the movement of muscles in the injured area; ③ they can stimulate the generation of blood vessels to restore nutrient supply. Specifically, Lee60 propagated human neuroblastoma (SH-SY5Y) cells on single graphene layer-coated slides and found increased neuronal processes in number and length, accompanied by increased expression of the neuronal marker like microtubule-associated protein 2 and Nestin. Moreover, FAK and P38 MAPK signaling pathways were thought to be involved in the regulation of neurite outgrowth and neuronal migration stimulated by graphene. In addition, using the electrical conduction properties of polylactic acid (PLA)/rGO3.5/polypyrrole (PPy) composite nanofibers, without additional growth factors, continuous electrical stimulation (400mV/cm) for 3 days can promote the adhesion, proliferation, and differentiation of rat adrenal pheochromocytoma (PC-12) cells into neural phenotype, as characterized by higher cell density and longer axons.61 Monolayer graphene-sodium alginate (GR-SA) hydrogel can simulate the extracellular matrix system, thus promoting the expression of myelin basic protein (MBP), nerve growth factor (NGF), and nerve growth associated protein-43 (GAP43), and inhibiting the secretion of pro-inflammatory factors such as IL-6 and TNF-α in rat Schwann cells. These changes have also been confirmed in the rat clamp injury model, as shown by highly expressed nerve growth factors, increased number and length of the axon, and better sciatic nerve functional index value (SFI) (demonstrated in Figure 2).12 In another rat clamp model, the implantation of polycaprolactone (PCL)-loaded graphene scaffolds significantly increased the expression of MBP and β-III-tubulin in the nerve tissue, accompanied by myelin sheath extension and axon outgrowth. The authors further pointed out that graphene could activate and regulate both Astrocytes and Schwann cells (SCs) to promote VEGF and fast myosin expression, leading to enhanced angiogenesis and muscle vitality, and the final recovery of electrophysiology and motor function in the hind limbs of rats.62
![]() |
Figure 2 The neuron morphology analysis of regenerated sciatic nerve after 4 weeks and functional analysis. (A–I) Transmission electron microscope (TEM) observation of regenerated sciatic nerve after 4 weeks. (J-L) HE staining pictures of the regenerated sciatic nerve. (M–O) TB staining pictures of the regenerated sciatic nerve. (P) Functional analysis of is of the thickness of myelin sheath. (R) Statistical analysis of the average diameter of myelinated axons. All data are presented as mean ± SD. **p < 0.01. Notes: Used with permission of Elsevier Science & Technology Journals. Y. Jin, W. Zhang, Y. Zhang, Y. Yang, Z. Fang, J. Song, Y. Qian, W. Yuan. Multifunctional biomimetic hydrogel based on graphene nanoparticles and sodium alginate for peripheral nerve injury therapy, Biomaterials Advances 135 (2022) 212727. Copyright 2022 Elsevier.12 |
Myocardial Tissue Repair and Reconstruction
Myocardial infarction (MI) is a common life-threatening heart disease that causes scarred tissue in the infarcted area, blocking cardiomyocytes’ normal electrical transduction signal and causing ventricular dysfunction. Restoring the electrical coupling between the infarct area and the surrounding tissue is an effective way to improve cardiac function. The good electrical conductivity and high mechanical strength of GFNs can differentiate stem cells into cardiomyocytes, indicating their great potential in myocardial tissue regeneration. For example, GO and rGO were reported to promote human umbilical cord-derived mesenchymal stem cells (hUC-MSCs) to differentiate into cardiomyocyte-like cells by activating PI3K/AKT/GSK pathway, promoting GATA binding protein 4 (GATA-4) expression.63 Furthermore, the addition of graphene film to PEG substrate material can improve the conductivity of the scaffold and effectively improve the electrophysiological function of cardiomyocytes. In cardiomyocytes, the expression of connexin 43 (Cx43) and sarcoplasmic reticulum Ca2+-ATPase were up-regulated, and the amplitude of calcium transient was increased, suggesting that graphene can promote the circulation of calcium ions in the cardiomyocyte’s sarcoplasmic reticulum, causing more calcium release during depolarization and finally prolonging the action potential plateau period and increasing action potential duration.14 A follow-up study found rGO dispersed in the decellularized extracellular matrices (dECM) of myocardial cells, making dECM-rGO hydrogels, can also promote differentiation of human induced pluripotent stem cells (hiPSCs) into mature and functional myocardial cells. In addition to increased expression of Cx43 protein, rGO also increased the cell depolarization rate and prolonged the action potential plateau phase by promoting the expression of calcium channels CACNA1C and ATP2A2 and potassium channels KCNH2 and KCNE1. Furthermore, rGO also enhances the hydrogel’s compressive strength, making its hardness similar to healthy myocardium, which is beneficial for enhancing the contractility of the cells.64 In the in vivo MI rats study, chitosan (CS) scaffolds containing GO-Au nanosheets were found to increase Cx43 protein level and intercellular coupling at the infarcted myocardium, leading to improved conduction efficiency and contractility. This effect is also linked with GO-mediated increased mechanical strength and decreased resistivity of the composite scaffolds.65
Importantly, GFNs can also increase the regenerative potential of cardiomyocytes through stimulating angiogenesis. Zhao et al implanted rGO/SilkR patch at the MI site in rats and observed increased left ventricular fractional shortening (LVFS) and ejection fractions, and improved ventricular pumping. Possible mechanisms include rGO-mediated ①increased expression of cardiac troponin T in cardiomyocytes and Cx43 in intercalated discs, ②promotion of CD31 expression to reduce the destruction and increase the formation of small microvessels, and ③inhibit the expression of α-SMA to reduce the formation of large microvessels, all together to improve the blood supply for the infarction area.10
Oral Tissue Regeneration
Pulp regeneration refers to the proliferation and differentiation of dental pulp stem cells (DPSCs) to form a pulpodentinal complex,66 which is used to treat pathological or iatrogenic pulp lesions caused by caries and pulpotomy, etc. Currently, an ideal material for pulp regeneration has not been developed; however, increasing evidence suggests that GFNs can be used to promote pulp regeneration. For instance, following GO treatment, a significant increase in the mRNA expression of odontoblastic genes including msh homeobox 1 (MSX-1), paired box 9 (PAX-9), dentin matrix acidic phosphoprotein 1 (DMP-1), dentin sialophosphoprotein (DSPP), and the osteogenic regulatory gene runt-related transcription factor 2 (RUNX2) were observed in DPSCs, suggesting that GO could induce both odontogenic and osteogenic differentiation of DPSCs.67 The authors further found that only osteogenic genes, such as RUNX2, collagen type I (COL I) and osteocalcin (OCN) were significantly up-regulated after graphene exposure, while odontoblastic genes were down-regulated.68 The above two studies indicated different potentials for promoting DPSCs differentiation between graphene and GO. Compared with pure mesoporous bioactive glass nanoparticle (MBN), MBN/GO composites significantly increased the mRNA and protein levels of odontogenic differentiation markers including DSPP, DMP-1, RUNX-2, and bone morphogenetic protein 2 (BMP-2) in hDPSCs via activating Wnt/β-catenin signaling pathway, enhancing intracellular ALP activity and mineralization capacity.69 GFNs can also promote the neurogenic differentiation of DPSCs. Seonwoo et al70 found that the early Tuj-1 and late NeuN markers of neurogenesis were highly expressed after rGO-PCL nanofibers treatment in DPSCs. Note that this effect is closely related to rGO doses, as 0.1 wt% rGO with neatly arranged nanofibers would promote nerve axon extension, while 1wt% rGO caused axon shortening and neurodegeneration of DPSCs. Consequently, it is confirmed that the administration dose is a major determinant of the biological character of GFNs during their utilization in tissue regeneration.
Periodontal ligament stem cells (PDLSCs) are the main functional cells that maintain periodontal tissue homeostasis and promote periodontal tissue regeneration through continuous proliferation and differentiation.71 Cultivation of PDLSCs on fibroin and graphene composites for 10 days resulted in enhanced cell proliferation and up-regulation of osteogenic genes, such as RUNX2, BMP-2, ALP, and osteogenic markers such as cementum attachment protein (CAP) and cementum protein 1 (CEMP1).72 Similar findings were also detected in PDLSCs following cultivation on GO-coated titanium matrix, suggesting that GO-coated modification may help improve osseointegration of clinical titanium implants, although further in vivo validation is still required.73 In an in vivo study, implantation of GO-3D collagen sponge scaffold in a rat’s skull defects induced a respective 3.7 and 3.3 times increase in the new bone’s area and height than single collagen group. This observation was also confirmed in the dog model of class II root bifurcation lesions, which revealed periodontal attachment formation after 4 weeks after the same treatment, including the regeneration of alveolar bone, periodontal ligament-like tissue, and dentinal tissue.74 Moreover, in the mouse orthodontic tooth movement model, local injection of gelatin-rGO (GOG) solution induced more osteoclasts in the compression side and more new bone formations in the tension side of the tooth. More blood vessels were also detected around the bone resorption and bone formation sites (demonstrated in Figure 3).75 These findings indicated that GOG could accelerate bone remodeling and orthodontic tooth movement through promotion on osteoblasto-/osteoclasto-genesis and angiogenesis.
![]() |
Figure 3 The micro-CT and histological examination on the orthodontic tooth movement in vivo showed the more active bone remodeling in GOG injection group. (a) The sagittal and transversal sections of the micro-CT showed the changes of the alveolar bone around the tooth. White arrow indicates the mesial periodontal membrane image which represents the activity of bone resorption and yellow arrow shows the delayed bone resorption in PBS group, white triangle indicates the distal periodontal membrane image which represents the activity of bone formation, bar: 500 μm. (b) The line profile of the gray values in the sagittal sections. The red and green dotted line indicates the mesial and distal periodontal membrane width of the root respectively. (c) The TRAP staining of the sections showed the more detected osteoclast activities (the purple stained position indicates the mature osteoclast) in GOG group compared with PBS group, bar: 500 μm. (d) The sequential fluorescence labeling images showed the activity level of the new bone formation, which was labeled through the intraperitoneal injection Alizarin Red S (AL) at 0 day and Calcein (CA) at 7 day after the establishment of OTM model, bar: 400 μm. (e) The quantitative analysis of the fluorochrome stained bone showed the increased bone formation in GOG group compared with PBS injection groups. *p < 0.05 indicates significant difference compared to PBS group. (f) The magnified field of the TRAP staining showed the detailed bone formation and resorption activities. The white arrow indicates the blood vessels and triangle indicates the mature osteoclast, yellow dotted line indicates the border between the new bone formation/bone resorption and the old bone structure, bar: 100 μm. (g) The HE staining also showed the similar condition of bone remodeling (bone formation, bone resorption and angiogenesis). The white arrow indicates the blood vessels, bar: 100 μm. Notes: Reproduced from D. Jiao, J. Wang, W. Yu, K. Zhang, N. Zhang, L. Cao, X. Jiang, Y. Bai. Biocompatible reduced graphene oxide stimulated BMSCs induce acceleration of bone remodeling and orthodontic tooth movement through promotion on osteoclastogenesis and angiogenesis, Bioactive Materials 15 (2022) 409–425. Creative Commons.75 |
Vascular Repair and Reconstruction
The formation of a functional vascular network is imperative for facilitating the regeneration of vascularized tissues and organs, including the heart, muscle, and kidney. However, studies have shown that angiogenic cells exhibit limited activity post-transplantation, leading to a notable decline in their angiogenic capabilities. These challenges exacerbate the complexities associated with tissue regeneration engineering.76,77 Currently, it has been documented that GFNs have the capability to stimulate the release of angiogenic factors, including VEGF and angiopoietin-1, from vascular endothelial cells or mesenchymal stem cells. This process subsequently triggers downstream signaling pathways that facilitate endothelial cell migration, ultimately promoting angiogenesis.78 In-vivo studies have found that after adding rGO to different components such as PVA/carboxymethyl cellulose79 or gelatin-methacryloyl hydrogels,8 the number and thickness of blood vessels in the chicken embryo chorioallantoic membrane notably increased, suggesting a satisfying pro-angiogenic potential of rGO. Similar to their utilization in oral tissue regeneration, GO at suitable concentrations demonstrated the ability to enhance the vascular formation potential of hydrogels. This was evidenced by the observation that CS hydrogel scaffolds supplemented with 0.1wt% and 0.5wt% GO facilitated the proliferation of endothelial progenitor cells and induced angiogenesis through the up-regulation of angiogenic genes such as VEGF, matrix metalloproteinase 9 (MMP 9), and stromal cell-derived factor 1 (SDF-1).80
A variety of endogenous signaling molecules participate in the regulation of VEGF expression. Specifically, following hypoxia-induced activation, hypoxia-inducible factor-1α (HIF-1α) translocates to the nucleus where it directly binds to the hypoxia response element on the VEGF promoter, thereby enhancing its transcription.81 In comparison to scaffolds made of pure mesoporous, bioactive glasses incorporating GO (MBG-GO) upregulated the mRNA levels of HIF-1α and VEGF in rat bone marrow mesenchymal stem cells. The number of newly formed blood vessels and bone tissue in the rats’ skull defects also increased significantly.82 Myogenic differentiation (MyoD) is also required to maintain proper levels of VEGF.83 Increased number of endothelial cell vascular nodes and endothelioid cells were respectively observed in HUVECs and peripheral blood vessels of chicken embryos after using the culture medium of PMC grown in GO scaffold.84 This finding resulted from GO-induced elevated expressions of MyoD1, which further promoted the synthesis and secretion of VEGF-A protein. More interestingly, GO could also stimulate VEGF secretion in mouse monocytes of macrophages (RAW264.7 cells), then activating the related pathway genes of HUVECs (including VEGFR1, VEGF2, platelet-derived growth factor receptor α (PDGFRα) and PDGFRβ) to promote vascularization.85
GFNs also regulate other angiogenic factors. For example, Jiao et al cultured bone marrow stromal cells in a medium containing gelatin-rGO (GOG) in the upper transwell chamber and cultured rat aortic rings and HUVECs in the lower chamber. They found that new capillaries in the aortic rings and vascular budding of HUVECs increased. However, this proangiogenic effect was significantly weakened after the addition of PERK inhibitor GSK2606414, indicating an important role of PERK activation in GOG’s proangiogenesis.75 CD31, also known as platelet endothelial cell adhesion molecule 1, is actively involved in forming endothelial cell junctions and angiogenesis. GO/polycaprolactone (PCL) was reported to increase the area of CD31+ and vascular-like structures in rats’ regenerated sciatic nerves. In addition, the phosphorylation levels of activation of phosphorylation of protein kinase B (AKT), endothelial nitric oxide synthase (eNOS), and VEGFR2 in neural tissues were significantly increased, suggesting that GO could stimulate the AKT-NOS pathway to promote VEGF expression and angiogenesis.86 Notably, low concentrations of ROS played a positive role in angiogenesis. GO and rGO could induce eNOS activation to produce NO and low levels of ROS, further to promote AKT phosphorylation, ultimately resulting in in-vivo and in-vitro angiogenesis.87 In 2022, it was also found that the binding of nano-graphene oxide to lysophosphatidic acid (LPA) in serum activates LPAR6 and promotes the formation of tip vascular endothelial cells and angiogenesis through the YAP signaling pathway, which is independent of intracellular ROS alterations (demonstrated in Figure 4).88 Collectively, Table 1 summarizes representative in vitro and in vivo studies of GFNs in regenerative medicine in recent years.
![]() |
Figure 4 NGO promoted the formation of new blood vessels in a rat model of calvarial bone defects. (a) NGO/GelMA nanocomposites were implanted into the defect area of rat calvarial bones. (b) HE staining of the defective region in the different groups 2 weeks after implantation. Scale bar, 100 μm. (c) CD31 immunohistochemical staining revealed newly formed blood vessels in the bone defect region 2 weeks after implantation. The results showed optimal lumen formation in the 0.5 wt% NGO/GelMA group (black arrows). Scale bar, 100 μm. (d) 3D images showing angiogenesis in the rat calvarial defect area after 2 weeks indicate adequate support and the best angiogenesis in the defective area in the group treated with the 0.5 wt% NGO/GelMA scaffold. (e) CD31 and Emcn IF staining revealed the presence of newly formed H-type blood vessels in the bone defect region (white arrows); the H-type blood vessel volume was quantified after 2 weeks. Data represent the mean ± SD (n = 6). Scale bars, 50 μm. **p < 0.005, ***p < 0.0001. Notes: Reproduced from W. Liu, H. Luo, Q. Wei, J. Liu, J. Wu, Y. Zhang, L. Chen, W. Ren, L. Shao. Electrochemically derived nanographene oxide activates endothelial tip cells and promotes angiogenesis by binding endogenous lysophosphatidic acid, Bioactive Materials 9 (2022) 92–104. Creative Commons.88 |
![]() |
Table 1 In vitro and in vivo Studies of GFNs in Regenerative Medicine |
Challenges and Perspectives
The unique physicochemical and biological properties of GFNs make them advantageous in regenerative medicine. However, a growing number of investigations have suggested that GFNs are not safe. They will cause potential toxic effects such as oxidative stress, inflammation, and hemolysis in biological systems, which severely limit their medical applications. For instance, it was reported that a low concentration (2.0 μg/mL) of GO resulted in significantly higher ROS production in two neuroblastoma cell lines SK-N-BE (2) and SH-SY5Y 24 hours after exposure.89 Incubation of human red blood cells in graphene quantum dots caused deformation and aggregation of red blood cells, and increased absorbance of hemoglobin, suggesting hemolysis.90 In-vivo, oral administration of GO nanoparticles caused damage to multiple organs throughout the body of Swiss mice, including apoptosis and inflammation of liver and brain tissue, and bone marrow cells DNA damage.91 GO could also penetrate the alveolar-capillary barrier of C57BL/6 female mice, stimulate the production of pro-inflammatory cytokines such as MCP-1 and IL-6, and cause lung inflammation.92
The GFNs toxicity is affected by their physicochemical properties. For example, primordial graphene accumulates on cell membranes and causes apoptosis via inducing oxidative stress. However, carboxylated hydrophilic graphene did not cause toxic reactions after internalization by cells.93 The use of PEG or bovine serum albumin (BSA) to coat GO and rGO surfaces significantly reduced DNA damage and cytotoxicity of pure nanomaterials in various cell lines, including human hepatocyte line HL-7702, lung fibroblast cell line MRC-5, and macrophage line U937.94 Furthermore, when bronchial epithelial (BEAS-2B) and alveolar epithelial (A549) cells were treated with different graphene derivatives, their viability was significantly lower than chemo-reduced GO (CRGO). This might be because GO has more oxidizing groups, which can lead to more severe intracellular oxidative stress.95 Akhavan et al reported that rGO nanosheets with smaller transverse size (11 ± 4 nm) have higher cytotoxicity than those with a larger transverse size (3.8 ± 0.4 μm).96 Additionally, disc-shaped GO showed better biocompatibility than polyhedral spherical graphene and ribbon-shaped GO as its materials had less interaction with cell membranes.97 Therefore, the biocompatibility of GFNs can be improved by increasing hydrophilicity, using biocompatible polymers to modify surfaces, reducing size and changing shape, etc. The graphene synthesis method is also an important factor affecting its biological behavior. Currently, in research, graphene and its derivatives used are in the form of a few controlled and uncontaminated defect-free samples, but the quality should be strictly controlled when used in clinical practice.98 Various unpredictable defects in the formation of GFNs are mainly due to differences in synthesis methods.99 Once a defect occurs, GFNs’s electronic structure, sensitivity, and reactivity will change.100 The conversion of graphene into GO requires a large number of strong oxidants; thus, GO is contaminated with impurities, which affects its biological properties. To improve the purity of GO, Mrózek et al washed the prepared GO multiple times with sodium chloride solution to obtain salt-washed graphene oxide (swGO) with higher purity and their toxicity to human embryonic lung fibroblasts (MRC-5) and human skin fibroblasts (HDF) was significantly reduced.101 Therefore, improving GFNs’s preparation method and the purity of nanomaterials is also a research direction that needs further exploration.
Other shortcomings also exist in the application of GFNs in regenerative medicine. Graphene coating of titanium implants can promote adhesion and osteogenic differentiation of human adipose-derived stem cells (hASCs) and bone marrow mesenchymal stem cells (hBMMSCs) and confer antibacterial activity on the titanium base. However, if the loading force exceeds 400 MPa, friction causes delamination of graphene coating in the titanium matrix.18 Future research needs to determine whether the shedding of the coating material can lead to chronic inflammation of the soft and hard tissues around the dental implant. Gu et al found that heat treatment at 160°C for 2h could enhance the bonding strength between graphene coating and titanium and did not affect the biological properties of graphene coating.102 Based on this, it is inferred that enhancing the bonding strength between graphene-based materials and titanium may be an effective method to reduce peri-implantitis and bone resorption after implant surgery.
GO is biodegradable by enzymes such as myeloperoxidase (MPO), horseradish peroxidase (HRP), and lignin peroxidase (LiP).103 Leon et al found that the degradation of GO sheets occurred in macrophages and neutrophils, which was related to the phagocytic function of the cells and the MPO expression.104 It has been suggested that materials used in bone tissue regeneration should be degradable. Furthermore, the slow degradation of scaffold materials is conducive to bone regeneration, providing enough time for new bone tissue to grow.105 By increasing GO concentration in gelatin-alginate-GO composite scaffolds, its physical cross-linking ability can be used to slow down the scaffolds’ biodegradation rate.19 The degradation rate could be also reduced by increasing the content of TiO2 in the TiO2/HAp/BG-PVA nanocomposite scaffold, which reduced the hydropathy of the scaffold.106 It is worth noting that good hydrophilicity promotes cell adhesion on scaffolds, thus a balance should be struck between a moderate degradation rate and good hydrophilicity. On the other side, the long-term presence of the scaffold in the body can actually compress the nerve after the repair and reconstruction of neural tissue is completed. Re-operation to remove the material will cause secondary injury to patients. Therefore, to match the rate of scaffold degradation with the rate of tissue regeneration, materials applied to neural tissue repair and reconstruction should have a relatively fast degradation rate.107 Yashaswini et al revealed that increased porosity of GO-containing composite scaffolds can increase their surface, providing more active sites for lysozyme reaction, thereby improving the degradation rate of the material.105 This provides a reference idea for future research on how to speed up the degradation of GO materials.
Recent studies have reported that GFNs also have the potential to promote ocular tissue regeneration. A variety of human eye cell lines and primary human cells can adhere, grow, and proliferate on rGO membrane (rGOM), maintain their characteristic morphology, and express characteristic markers, including zonula occludens-1 (ZO-1), RPE65, E-cadherin, etc. Additionally, rGOM implantation in the corneal stroma or intrascleral does not cause tissue inflammation or damage to the integrity of the cornea and retina.20 Jia et al applied inkjet printing technology to control GO deposition on the microelectrode surface and observed that human retinal pigment epithelial (RPE) cells specifically adhered to the micropatterned GO surface on the chip and demonstrated good ZO-1 expression. It is suggested that the attached cells still function as subretinal chips and are expected to fully communicate with the remaining retinal cells, thereby restoring visual perception.21 The above studies show that GFNs have good biocompatibility with ocular tissues, indicating a great potential in ocular tissue engineering and treatment. However, there is currently limited research in this area, and further exploration is required for its biological mechanism and application prospects in promoting ocular tissue regeneration.
Summary
GFNs are a novel class of carbon-based 2D nanomaterials that exhibit notable physicochemical properties such as exceptional mechanical strength, chemical stability, porous structure, and favorable hydrophilicity. These inherent characteristics have been found to augment their biological properties by facilitating cellular adhesion, proliferation, and differentiation. Moreover, GFNs have been shown to create an anti-inflammatory milieu, exert antibacterial effects, and possess excellent electrical conductivity, thereby presenting substantial advantages in their potential applications for bone and oral tissue (including tooth tissue) regeneration, and repairing of nerve, myocardium, and blood vessels. This review details the biological basis, application status, and current challenges of GFNs in regenerative medicine, which helps to understand their key molecular mechanism and signaling targets that promote tissue regeneration and repair. More importantly, we hope to provide a reference for using GFNs to treat related clinical diseases including jaw defects, nerve damage, periodontitis, and atherosclerotic cardiovascular diseases.
Acknowledgment
The graphical abstract was created with BioRender.com.
Author Contributions
All authors made a significant contribution to the work reported, whether that is in the conception, study design, execution, acquisition of data, analysis and interpretation, or in all these areas; took part in drafting, revising or critically reviewing the article; gave final approval of the version to be published; have agreed on the journal to which the article has been submitted; and agree to be accountable for all aspects of the work.
Funding
This work was supported by the National Natural Science Foundation of China (82001298), Science research cultivation program of stomatological hospital, Southern medical university (PY2023020), Science and Technology Projects in Guangzhou (2023A04J2396), Outstanding Youths Development Scheme of Nanfang Hospital, Southern Medical University (2022J004), Guangdong Basic and Applied Basic Research Foundation (2019A1515110635), Medical Scientific Research Foundation of Guangdong Province of China (A2020131).
Disclosure
The authors declare that they have no conflicts of interest.
References
1. Omran B, Baek KH. Graphene-derived antibacterial nanocomposites for water disinfection: current and future perspectives. Environ Pollut. 2022;298:118836. doi:10.1016/j.envpol.2022.118836
2. Xu GQ, Xu PW, Shi DJ, Chen MQ. Preparation and Cellular Imaging of PEG Grafted Graphene Oxide. Chinese J Inorg Chem. 2014;30(09):1994–1999.
3. Gu YM. Study of Polyamidoamine Modified Graphene Oxide as Drug and Gene or Drug and Drug Co-Delivery Carrier. Jinan University; 2017.
4. Kulshrestha S, Khan S, Meena R, Singh BR, Khan AU. A graphene/zinc oxide nanocomposite film protects dental implant surfaces against cariogenic Streptococcus mutans. Biofouling. 2014;30(10):1281–1294. doi:10.1080/08927014.2014.983093
5. Khalili R, Zarrintaj P, Jafari SH, Vahabi H, Saeb MR. Electroactive poly (p-phenylene sulfide)/r-graphene oxide/chitosan as a novel potential candidate for tissue engineering. Int J Biol Macromol. 2020;154:18–24. doi:10.1016/j.ijbiomac.2020.03.029
6. Wang YQ, Yu HR, Liu HF, Fan YB. Double coating of graphene oxide–polypyrrole on silk fibroin scaffolds for neural tissue engineering. J Bioact Compat Pol. 2020;35(3):216–227. doi:10.1177/0883911520913905
7. Wang XT, Guo WM, Li LY, et al. Photothermally triggered biomimetic drug delivery of Teriparatide via reduced graphene oxide loaded chitosan hydrogel for osteoporotic bone regeneration. Chem Eng J. 2021;413(127413). doi:10.1016/j.cej.2020.127413
8. Rehman S, Augustine R, Zahid AA, Ahmed R, Tariq M, Hasan A. Reduced graphene oxide incorporated GelMA hydrogel promotes angiogenesis for wound healing applications. Int J Nanomed. 2019;14:9603–9617. doi:10.2147/IJN.S218120
9. Fang X, Guo H, Zhang W, et al. Reduced graphene oxide-GelMA-PCL hybrid nanofibers for peripheral nerve regeneration. J Mater Chem B. 2020;8(46):10593–10601. doi:10.1039/d0tb00779j
10. Zhao G, Feng Y, Xue L, et al. Anisotropic conductive reduced graphene oxide/silk matrices promote post-infarction myocardial function by restoring electrical integrity. Acta Biomater. 2022;139:190–203. doi:10.1016/j.actbio.2021.03.073
11. Zhong L, Liu H, Samal M, Yun K. Synthesis of ZnO nanoparticles-decorated spindle-shaped graphene oxide for application in synergistic antibacterial activity. J Photochem Photobiol B. 2018;183:293–301. doi:10.1016/j.jphotobiol.2018.04.048
12. Jin Y, Zhang WK, Zhang YH, et al. Multifunctional biomimetic hydrogel based on graphene nanoparticles and sodium alginate for peripheral nerve injury therapy. Biomat Advan. 2022;135:212727. doi:10.1016/j.bioadv.2022.212727
13. Sun J, Li L, Xing F, et al. Graphene oxide-modified silk fibroin/nanohydroxyapatite scaffold loaded with urine-derived stem cells for immunomodulation and bone regeneration. Stem Cell Res Ther. 2021;12(1):591. doi:10.1186/s13287-021-02634-w
14. Smith A, Yoo H, Yi H, et al. Micro- and nano-patterned conductive graphene-PEG hybrid scaffolds for cardiac tissue engineering. Chem Commun. 2017;53(53):7412–7415. doi:10.1039/c7cc01988b
15. Mu R. LIM Mineralized Protein 1 Regulates Human Pulp Stem Cells Combined With Graphene Oxide Modified Scaffolds to Promote Tissue Regeneration. Southern Medical University; 2022.
16. Li R. Study on Drug delivery System of Nerve Repair with Electrical Stimulation and Angiogenesis. Wuhan University of Technology; 2020.
17. Xiaoli F, Qiyue C, Weihong G, et al. Toxicology data of graphene-family nanomaterials: an update. Arch Toxicol. 2020;94(6):1915–1939. doi:10.1007/s00204-020-02717-2
18. He R, Peng H, Liu F, et al. Crack initiation mechanism and life prediction of ti60 titanium alloy considering stress ratios effect in very high cycle fatigue regime. Materials. 2022;15(8). doi:10.3390/ma15082800
19. Purohit SD, Bhaskar R, Singh H, Yadav I, Gupta MK, Mishra NC. Development of a nanocomposite scaffold of gelatin-alginate-graphene oxide for bone tissue engineering. Int J Biol Macromol. 2019;133:592–602. doi:10.1016/j.ijbiomac.2019.04.113
20. Zambrano-Andazol I, Vázquez N, Chacón M, et al. Reduced graphene oxide membranes in ocular regenerative medicine. Mater Sci Eng C Mater Biol Appl. 2020;114:111075. doi:10.1016/j.msec.2020.111075
21. Yang JW, Tseng ML, Fu YM, et al. Printable graphene oxide micropatterns for a bio-subretinal chip. Adv Healthc Mater. 2018;7(18):e1800365. doi:10.1002/adhm.201800365
22. Kanayama I, Miyaji H, Takita H, et al. Comparative study of bioactivity of collagen scaffolds coated with graphene oxide and reduced graphene oxide. Int J Nanomed. 2014;9:3363–3373. doi:10.2147/IJN.S62342
23. Wang W. Effects of Graphene Oxide on Osteogenic Differentiation and Repairing Bone Defects. Shanghai Jiao Tong University; 2020.
24. Wang X, Li P, Wang Z, et al. Three-dimensional tissue engineering scaffolds based on graphene. Chin J Tissue Eng Res. 2015;19(34):5523–5529.
25. Liu S, Li Z, Wang Q, et al. Graphene Oxide/Chitosan/hydroxyapatite composite membranes enhance osteoblast adhesion and guided bone regeneration. ACS Appl Bio Mater. 2021;4(11):8049–8059. doi:10.1021/acsabm.1c00967
26. Wang GQ, Pang SQ, Lin JS, Diao Y. Research progress of graphene and its derivatives biomedicine field. J Huaq Univer. 2018;39(06):787–793.
27. Li XA, Wang BL, Liu ZR. Research progress in preparation, characterization and properties of graphene. Mater Rep. 2012;26(01):61–65.
28. Hu Y, Chen Z, Wang H, et al. Conductive nerve guidance conduits based on morpho butterfly wings for peripheral nerve repair. Acs Nano. 2022;16(2):1868–1879. doi:10.1021/acsnano.1c11627
29. Wang G, Zhang Y, Zhao S, et al. Graphene hollow micropatterns via capillarity-driven assembly for drug storage and neural cell alignment. ACS Appl Mater Interfaces. 2023;15(31):37775–37783. doi:10.1021/acsami.3c04217
30. Ghovvati M, Kharaziha M, Ardehali R, Annabi N. Recent advances in designing electroconductive biomaterials for cardiac tissue engineering. Adv Healthc Mater. 2022;11(13):e2200055. doi:10.1002/adhm.202200055
31. Bahrami S, Baheiraei N, Mohseni M, et al. Three-dimensional graphene foam as a conductive scaffold for cardiac tissue engineering. J Biomater Appl. 2019;34(1):74–85. doi:10.1177/0885328219839037
32. Zhang Y, Zhang M, Cheng D, et al. Applications of electrospun scaffolds with enlarged pores in tissue engineering. Biomater Sci. 2022;10(6):1423–1447. doi:10.1039/d1bm01651b
33. Newby SD, Masi T, Griffin CD, et al. Functionalized graphene nanoparticles induce human mesenchymal stem cells to express distinct extracellular matrix proteins mediating osteogenesis. Int J Nanomed. 2020;15:2501–2513. doi:10.2147/IJN.S245801
34. Guo R, Li J, Chen C, et al. Biomimetic 3D bacterial cellulose-graphene foam hybrid scaffold regulates neural stem cell proliferation and differentiation. Colloids Surf B Biointerfaces. 2021;200:111590. doi:10.1016/j.colsurfb.2021.111590
35. Èlia B, Leire D, Jenifer OB, Emilio C, Roman AP. Angiogenic and immunomodulation role of ions for initial stages of bone tissue regeneration. Acta Biomater. 2023;166:14–41. doi:10.1016/j.actbio.2023.06.001
36. Chen YN, Hu MR, Wang L, Chen WD. Macrophage M1/M2 polarization. Eur J Pharmacol. 2020;877:173090. doi:10.1016/j.ejphar.2020.173090
37. Han J, Kim YS, Lim MY, et al. Dual roles of graphene oxide to attenuate inflammation and elicit timely polarization of macrophage phenotypes for cardiac repair. Acs Nano. 2018;12(2):1959–1977. doi:10.1021/acsnano.7b09107
38. Hoyle C, Rivers-Auty J, Lemarchand E, et al. Small, thin graphene oxide is anti-inflammatory activating nuclear factor erythroid 2-related factor 2 via metabolic reprogramming. Acs Nano. 2018;12(12):11949–11962. doi:10.1021/acsnano.8b03642
39. Zhang Z, Zhao X, Wang C, Huang Y, Han Y, Guo B. Injectable conductive micro-cryogel as a muscle stem cell carrier improves myogenic proliferation, differentiation and in situ skeletal muscle regeneration. Acta Biomater. 2022;151:197–209. doi:10.1016/j.actbio.2022.08.036
40. Akhavan O, Ghaderi E, Esfandiar A. Wrapping bacteria by graphene nanosheets for isolation from environment, reactivation by sonication, and inactivation by near-infrared irradiation. J Phys Chem A. 2011;115(19):6279–6288. doi:10.1021/jp200686k
41. Akhavan O, Ghaderi E. Toxicity of graphene and graphene oxide nanowalls against bacteria. Acs Nano. 2010;4(10):5731–5736. doi:10.1021/nn101390x
42. Liu XT, Chen KL. Interactions of graphene oxide with model cell membranes: probing nanoparticle attachment and lipid bilayer disruption. Langmuir. 2015;31(44):12076–12086. doi:10.1021/acs.langmuir.5b02414
43. Wang X, Zhao W, Zhao C, Zhang W, Yan Z. Graphene coated Ti-6Al-4V exhibits antibacterial and antifungal properties against oral pathogens. J Prosthodont. 2023;32(6):505–511. doi:10.1111/jopr.13595
44. Chen J, Zhang X, Cai H, et al. Osteogenic activity and antibacterial effect of zinc oxide/carboxylated graphene oxide nanocomposites: preparation and in vitro evaluation. Colloids Surf B Biointerfaces. 2016;147:397–407. doi:10.1016/j.colsurfb.2016.08.023
45. Song B, Zhang C, Zeng GM, Gong JL, Chang YN, Jiang Y. Antibacterial properties and mechanism of graphene oxide-silver nanocomposites as bactericidal agents for water disinfection. Arch Biochem Biophys. 2016;604:167–176. doi:10.1016/j.abb.2016.04.018
46. Deng CH, Gong JL, Zhang P, Zeng GM, Song B, Liu HY. Preparation of melamine sponge decorated with silver nanoparticles-modified graphene for water disinfection. J Colloid Interf Sci. 2017;488:26–38. doi:10.1016/j.jcis.2016.10.078
47. Sun J, Liu X, Lv C, et al. Synergistic antibacterial effect of graphene-coated titanium loaded with levofloxacin. Colloids Surf B Biointerfaces. 2021;208:112090. doi:10.1016/j.colsurfb.2021.112090
48. Wen SL. Chemically Modified Graphene for Surface Modification of Three-Dimensional Porous Chitosan Scaffolds. Jilin University; 2017.
49. Chuan F. Application of Gold Nanoparticles Loaded Polydopamine Coating Modified Graphene Oxide Composite Material in Bone Defect Repair. Jilin University; 2021.
50. Zhou K, Yu P, Shi X, et al. Hierarchically porous hydroxyapatite hybrid scaffold incorporated with reduced graphene oxide for rapid bone ingrowth and repair. Acs Nano. 2019;13(8):9595–9606. doi:10.1021/acsnano.9b04723
51. Zhang L, Wang ZP, Xu C, et al. High strength graphene oxide/polyvinyl alcohol composite hydrogels. J Mater Chem. 2011;21(28):10399–10406. doi:10.1039/c0jm04043f
52. Liu J, Song G, He C, Wang H. Self-healing in tough graphene oxide composite hydrogels. Macromol Rapid Commun. 2013;34(12):1002–1007. doi:10.1002/marc.201300242
53. Fan P, Chen XP, Huang FL, Chen F, Yang JT, Zhong MQ. Preparation, structure and swelling behavior of Poly(N-isopropylacrylamide)/graphene nanocomposite hydrogel. J Mater Sci Eng. 2015;33(01):22–25+40. doi:10.14136/j.cnki.issn1673-2812.2015.01.006
54. Shi K, Liu Z, Wei YY, et al. Near-infrared light-responsive Poly(N-isopropylacrylamide)/graphene oxide nanocomposite hydrogels with ultrahigh tensibility. Acs Appl Mater Inter. 2015;7(49):27289–27298. doi:10.1021/acsami.5b08609
55. Wu M, Zou L, Jiang L, Zhao Z, Liu J. Osteoinductive and antimicrobial mechanisms of graphene-based materials for enhancing bone tissue engineering. J Tissue Eng Regen Med. 2021;15(11):915–935. doi:10.1002/term.3239
56. Tabatabaee S, Baheiraei N, Salehnia M. Fabrication and characterization of PHEMA-gelatin scaffold enriched with graphene oxide for bone tissue engineering. J Orthop Surg Res. 2022;17(1):216. doi:10.1186/s13018-022-03122-4
57. Khan M, Abd RS, Mehboob H, et al. Synthesis and characterization of silver-coated polymeric scaffolds for bone tissue engineering: antibacterial and in vitro evaluation of cytotoxicity and biocompatibility. ACS Omega. 2021;6(6):4335–4346. doi:10.1021/acsomega.0c05596
58. Maleki-Ghaleh H, Siadati MH, Fallah A, et al. Antibacterial and cellular behaviors of novel zinc-doped hydroxyapatite/graphene nanocomposite for bone tissue engineering. Int J Mol Sci. 2021;22(17). doi:10.3390/ijms22179564
59. Li Y, Yang L, Hou Y, et al. Polydopamine-mediated graphene oxide and nanohydroxyapatite-incorporated conductive scaffold with an immunomodulatory ability accelerates periodontal bone regeneration in diabetes. Bioact Mater. 2022;18:213–227. doi:10.1016/j.bioactmat.2022.03.021
60. Lee JS, Lipatov A, Ha L, et al. Graphene substrate for inducing neurite outgrowth. Biochem Biophys Res Commun. 2015;460(2):267–273. doi:10.1016/j.bbrc.2015.03.023
61. Liu RT. Preparation of Conductive Composites and Application in Tissue Engineering Under Electrical Stimulation. Guangdong University of Technology; 2021.
62. Qian Y, Wang X, Song JL, et al. Preclinical assessment on neuronal regeneration in the injury-related microenvironment of graphene-based scaffolds. npj Regenerat Med. 2021;6(1):31. doi:10.1038/s41536-021-00142-2
63. Sekuła-Stryjewska M, Noga S, Dźwigońska M, et al. Graphene-based materials enhance cardiomyogenic and angiogenic differentiation capacity of human mesenchymal stem cells in vitro - Focus on cardiac tissue regeneration. Mater Sci Eng C Mater Biol Appl. 2021;119:111614. doi:10.1016/j.msec.2020.111614
64. Tsui JH, Leonard A, Camp ND, et al. Tunable electroconductive decellularized extracellular matrix hydrogels for engineering human cardiac microphysiological systems. Biomaterials. 2021;272:120764. doi:10.1016/j.biomaterials.2021.120764
65. Saravanan S, Sareen N, Abu-El-Rub E, et al. Graphene oxide-gold nanosheets containing chitosan scaffold improves ventricular contractility and function after implantation into infarcted heart. Sci Rep-Uk. 2018;8(1). doi:10.1038/s41598-018-33144-0
66. Itoh Y, Sasaki JI, Hashimoto M, Katata C, Hayashi M, Imazato S. Pulp Regeneration by 3-dimensional dental pulp stem cell constructs. J Dent Res. 2018;97(10):1137–1143. doi:10.1177/0022034518772260
67. Rosa V, Xie H, Dubey N, et al. Graphene oxide-based substrate: physical and surface characterization, cytocompatibility and differentiation potential of dental pulp stem cells. Dent Mater. 2016;32(8):1019–1025. doi:10.1016/j.dental.2016.05.008
68. Xie H, Chua M, Islam I, et al. CVD-grown monolayer graphene induces osteogenic but not odontoblastic differentiation of dental pulp stem cells. Dent Mater. 2017;33(1):e13–e21. doi:10.1016/j.dental.2016.09.030
69. Ahn JH, Kim I, Kim Y, et al. The Effect of Mesoporous Bioactive Glass Nanoparticles/Graphene Oxide Composites on the Differentiation and Mineralization of Human Dental Pulp Stem Cells. Nanomaterials-Basel. 2020;10(4):620. doi:10.3390/nano10040620
70. Seonwoo H, Jang K, Lee D, et al. Neurogenic differentiation of human dental pulp stem cells on graphene-polycaprolactone hybrid nanofibers. Nanomaterials-Basel. 2018;8(7):554. doi:10.3390/nano8070554
71. Heng BC, Ye X, Liu Y, Dissanayaka WL, Cheung GSP, Zhang C. Effects of recombinant overexpression of Bcl2 on the proliferation, apoptosis, and osteogenic/odontogenic differentiation potential of dental pulp stem cells. J Endod. 2016;42(4):575–583. doi:10.1016/j.joen.2016.01.013
72. Vera-Sánchez M, Aznar-Cervantes S, Jover E, et al. Silk-fibroin and graphene oxide composites promote human periodontal ligament stem cell spontaneous differentiation into osteo/cementoblast-like cells. Stem Cells Dev. 2016;25(22):1742–1754. doi:10.1089/scd.2016.0028
73. Zhou Q, Yang PS, Li XL, Liu H, Ge SH. Bioactivity of periodontal ligament stem cells on sodium titanate coated with graphene oxide. Sci Rep-Uk. 2016;6(1). doi:10.1038/srep19343
74. Kawamoto K, Miyaji H, Nishida E, et al. Characterization and evaluation of graphene oxide scaffold for periodontal wound healing of class II furcation defects in dog. Int J Nanomed. 2018;13:2365–2376. doi:10.2147/IJN.S163206
75. Jiao DL, Wang J, Yu WT, et al. Biocompatible reduced graphene oxide stimulated BMSCs induce acceleration of bone remodeling and orthodontic tooth movement through promotion on osteoclastogenesis and angiogenesis. Bioact Mater. 2022;15:409–425. doi:10.1016/j.bioactmat.2022.01.021
76. Kocijan T, Rehman M, Colliva A, et al. Genetic lineage tracing reveals poor angiogenic potential of cardiac endothelial cells. Cardiovasc Res. 2021;117(1):256–270. doi:10.1093/cvr/cvaa012
77. Chong MSK, Ng WK, Chan JKY. Concise Review: endothelial progenitor cells in regenerative medicine: applications and challenges. Stem Cells Transl Med. 2016;5(4):530–538. doi:10.5966/sctm.2015-0227
78. Li T, Zhang T. The application of nanomaterials in angiogenesis. Curr Stem Cell Res Ther. 2021;16(1):74–82. doi:10.2174/1574888X15666200211102203
79. Chakraborty S, Ponrasu T, Chandel S, Dixit M, Muthuvijayan V. Reduced graphene oxide-loaded nanocomposite scaffolds for enhancing angiogenesis in tissue engineering applications. R Soc Open Sci. 2018;5(5):172017. doi:10.1098/rsos.172017
80. Zhang LF, Li XP, Shi CY, et al. Biocompatibility and angiogenic effect of chitosan/graphene oxide hydrogel scaffolds on EPCs. Stem Cells Int. 2021;2021:1–17. doi:10.1155/2021/5594370
81. Tan W, Jia W, Sun V, Mihm MJ, Nelson JS. Topical rapamycin suppresses the angiogenesis pathways induced by pulsed dye laser: molecular mechanisms of inhibition of regeneration and revascularization of photocoagulated cutaneous blood vessels. Lasers Surg Med. 2012;44(10):796–804. doi:10.1002/lsm.22101
82. Wang W, Liu Y, Yang C, et al. Mesoporous bioactive glass combined with graphene oxide scaffolds for bone repair. Int J Biol Sci. 2019;15(10):2156–2169. doi:10.7150/ijbs.35670
83. Bryan BA, Walshe TE, Mitchell DC, et al. Coordinated vascular endothelial growth factor expression and signaling during skeletal myogenic differentiation. Mol Biol Cell. 2008;19(3):994–1006. doi:10.1091/mbc.e07-09-0856
84. Wierzbicki M, Hotowy A, Kutwin M, et al. Graphene oxide scaffold stimulates differentiation and proangiogenic activities of myogenic progenitor cells. Int J Mol Sci. 2020;21(11):4173. doi:10.3390/ijms21114173
85. Xue D, Chen E, Zhong H, et al. Immunomodulatory properties of graphene oxide for osteogenesis and angiogenesis. Int J Nanomed. 2018;13:5799–5810. doi:10.2147/IJN.S170305
86. Qian Y, Song JL, Zhao XT, et al. 3D fabrication with integration molding of a graphene oxide/polycaprolactone nanoscaffold for neurite regeneration and angiogenesis. Adv Sci. 2018;5(4):1700499. doi:10.1002/advs.201700499
87. Mukherjee S, Sriram P, Barui AK, et al. Graphene oxides show angiogenic properties. Adv Healthc Mater. 2015;4(11):1722–1732. doi:10.1002/adhm.201500155
88. Liu WJ, Luo HY, Wei QW, et al. Electrochemically derived nanographene oxide activates endothelial tip cells and promotes angiogenesis by binding endogenous lysophosphatidic acid. Bioact Mater. 2022;9:92–104. doi:10.1016/j.bioactmat.2021.07.007
89. Mari E, Mardente S, Morgante E, et al. Graphene oxide nanoribbons induce autophagic vacuoles in neuroblastoma cell lines. Int J Mol Sci. 2016;17(12):1995. doi:10.3390/ijms17121995
90. Kim J, Nafiujjaman M, Nurunnabi M, Lee Y, Park H. Hemorheological characteristics of red blood cells exposed to surface functionalized graphene quantum dots. Food Chem Toxicol. 2016;97:346–353. doi:10.1016/j.fct.2016.09.034
91. Mohamed H, Welson M, Yaseen AE, El-Ghor A. Induction of chromosomal and DNA damage and histological alterations by graphene oxide nanoparticles in Swiss mice. Drug Chem Toxicol. 2021;44(6):631–641. doi:10.1080/01480545.2019.1643876
92. Duch MC, Budinger GRS, Liang YT, et al. Minimizing oxidation and stable nanoscale dispersion improves the biocompatibility of graphene in the lung. Nano Lett. 2011;11(12):5201–5207. doi:10.1021/nl202515a
93. Sasidharan A, Panchakarla LS, Chandran P, et al. Differential nano-bio interactions and toxicity effects of pristine versus functionalized graphene. Nanoscale. 2011;3(6):2461–2464. doi:10.1039/c1nr10172b
94. Li Y, Feng L, Shi X, et al. Surface coating-dependent cytotoxicity and degradation of graphene derivatives: towards the design of non-toxic, degradable nano-graphene. Small. 2014;10(8):1544–1554. doi:10.1002/smll.201303234
95. Mittal S, Kumar V, Dhiman N, Chauhan LKS, Pasricha R, Pandey AK. Physico-chemical properties based differential toxicity of graphene oxide/reduced graphene oxide in human lung cells mediated through oxidative stress. Sci Rep-Uk. 2016;6(1). doi:10.1038/srep39548
96. Akhavan O, Ghaderi E, Akhavan A. Size-dependent genotoxicity of graphene nanoplatelets in human stem cells. Biomaterials. 2012;33(32):8017–8025. doi:10.1016/j.biomaterials.2012.07.040
97. Talukdar Y, Rashkow JT, Lalwani G, Kanakia S, Sitharaman B. The effects of graphene nanostructures on mesenchymal stem cells. Biomaterials. 2014;35(18):4863–4877. doi:10.1016/j.biomaterials.2014.02.054
98. Skoda M, Dudek I, Jarosz A, Szukiewicz D. Graphene: one material, many possibilities-application difficulties in biological systems. J Nanomater. 2014;2014(890246). doi:10.1155/2014/890246
99. Rodríguez-Pérez L, Herranz MÁ, Martín N. The chemistry of pristine graphene. Chem Commun. 2013;49(36):3721–3735. doi:10.1039/c3cc38950b
100. Gao X, Wang Y, Liu X, et al. Regioselectivity control of graphene functionalization by ripples. Phys Chem Chem Phys. 2011;13(43):19449–19453. doi:10.1039/c1cp22491c
101. Mrózek O, Melounková L, Smržová D, et al. Salt-washed graphene oxide and its cytotoxicity. J Hazard Mater. 2020;398:123114. doi:10.1016/j.jhazmat.2020.123114
102. Gu M, Lv LW, Du F, et al. Effects of thermal treatment on the adhesion strength and osteoinductive activity of single-layer graphene sheets on titanium substrates. Sci Rep-Uk. 2018;8(1). doi:10.1038/s41598-018-26551-w
103. Daneshmandi L, Barajaa M, Tahmasbi RA, Sydlik SA, Laurencin CT. Graphene-based biomaterials for bone regenerative engineering: a comprehensive review of the field and considerations regarding biocompatibility and biodegradation. Adv Healthc Mater. 2021;10(1):e2001414. doi:10.1002/adhm.202001414
104. Newman L, Jasim DA, Prestat E, et al. Splenic capture and in vivo intracellular biodegradation of biological-grade graphene oxide sheets. Acs Nano. 2020;14(8):10168–10186. doi:10.1021/acsnano.0c03438
105. Devi GVY, Nagendra AH, Shenoy PS, Chatterjee K, V J. Fucoidan-incorporated composite scaffold stimulates osteogenic differentiation of mesenchymal stem cells for bone tissue engineering. Mar Drugs. 2022;20(10). doi:10.3390/md20100589
106. Aslam KM, Al-Arjan WS, Binkadem MS, et al. Development of biopolymeric hybrid scaffold-based on aac/go/nhap/tio(2) nanocomposite for bone tissue engineering: in-vitro analysis. Nanomaterials. 2021;11(5). doi:10.3390/nano11051319
107. Yao RZ, Wang BW, Wang GL. Research progress of graphene and its derivatives in repair of peripheral nerve defect. Chin J Reparative Reconst Surg. 2018;32(11):1483–1487. doi:10.7507/1002-1892.201804096
© 2024 The Author(s). This work is published and licensed by Dove Medical Press Limited. The
full terms of this license are available at https://www.dovepress.com/terms.php
and incorporate the Creative Commons Attribution
- Non Commercial (unported, v3.0) License.
By accessing the work you hereby accept the Terms. Non-commercial uses of the work are permitted
without any further permission from Dove Medical Press Limited, provided the work is properly
attributed. For permission for commercial use of this work, please see paragraphs 4.2 and 5 of our Terms.