Back to Journals » International Journal of Nanomedicine » Volume 19
Strategies and Recent Advances on Improving Efficient Antitumor of Lenvatinib Based on Nanoparticle Delivery System
Authors Wang H, Bo W, Feng X, Zhang J, Li G, Chen Y
Received 23 January 2024
Accepted for publication 28 May 2024
Published 10 June 2024 Volume 2024:19 Pages 5581—5603
DOI https://doi.org/10.2147/IJN.S460844
Checked for plagiarism Yes
Review by Single anonymous peer review
Peer reviewer comments 2
Editor who approved publication: Dr Kamakhya Misra
Haiqing Wang,1,* Wentao Bo,1,* Xielin Feng,1 Jinliang Zhang,1 Ge Li,2 Yan Chen3
1Department of Hepatopancreatobiliary Surgery, Sichuan Clinical Research Center for Cancer, Sichuan Cancer Hospital and Institute, Affiliated Cancer Hospital of University of Electronic Science and Technology of China, Chengdu, People’s Republic of China; 2Department of Emergency, Sichuan Clinical Research Center for Cancer, Sichuan Cancer Hospital and Institute, Affiliated Cancer Hospital of University of Electronic Science and Technology of China, Chengdu, People’s Republic of China; 3Department of Pharmacy, Sichuan Clinical Research Center for Cancer, Sichuan Cancer Hospital and Institute, Affiliated Cancer Hospital of University of Electronic Science and Technology of China, Chengdu, People’s Republic of China
*These authors contributed equally to this work
Correspondence: Yan Chen; Ge Li, Email [email protected]; [email protected]; [email protected]
Abstract: Lenvatinib (LVN) is a potentially effective multiple-targeted receptor tyrosine kinase inhibitor approved for treating hepatocellular carcinoma, metastatic renal cell carcinoma and thyroid cancer. Nonetheless, poor pharmacokinetic properties including poor water solubility and rapid metabolic, complex tumor microenvironment, and drug resistance have impeded its satisfactory therapeutic efficacy. This article comprehensively reviews the uses of nanotechnology in LVN to improve antitumor effects. With the characteristic of high modifiability and loading capacity of the nano-drug delivery system, an active targeting approach, controllable drug release, and biomimetic strategies have been devised to deliver LVN to target tumors in sequence, compensating for the lack of passive targeting. The existing applications and advances of LVN in improving therapeutic efficacy include improving longer-term efficiency, achieving higher efficiency, combination therapy, tracking and diagnosing application and reducing toxicity. Therefore, using multiple strategies combined with photothermal, photodynamic, and immunoregulatory therapies potentially overcomes multi-drug resistance, regulates unfavorable tumor microenvironment, and yields higher synergistic antitumor effects. In brief, the nano-LVN delivery system has brought light to the war against cancer while at the same time improving the antitumor effect. More intelligent and multifunctional nanoparticles should be investigated and further converted into clinical applications in the future.
Keywords: lenvatinib, nano-drug delivery system, antitumor, side effects, diagnosis
Graphical Abstract:
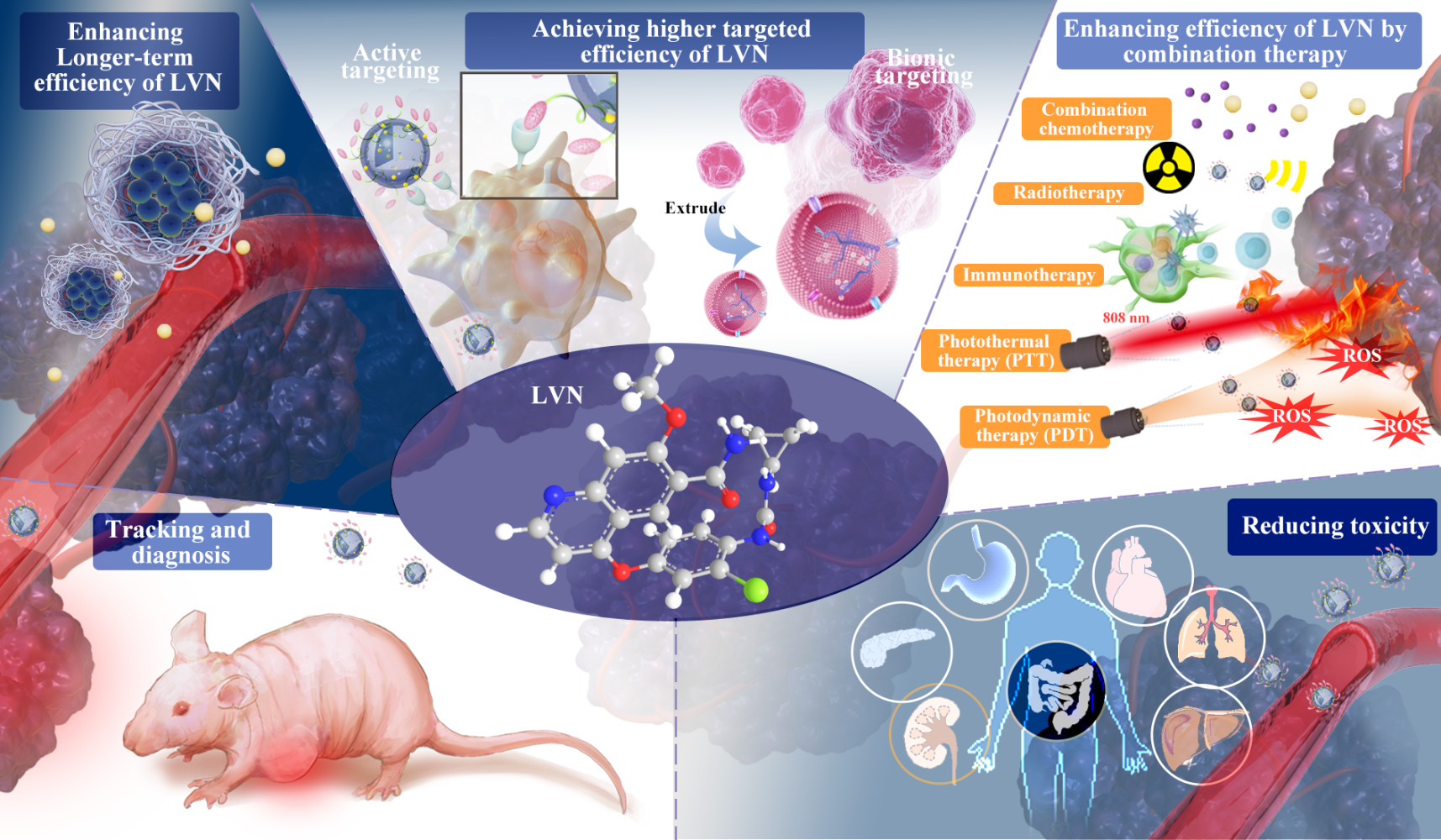
Introduction
Cancer remains the most common disease and one of the leading causes of death due to a high incidence and late diagnosis. The commonly used treatment methods include surgery, radiotherapy, chemotherapy, targeting therapy, and immunotherapy. With advances in targeted therapeutics, survival rates have significantly improved for numerous advanced-stage cancers, including hepatocellular carcinoma (HCC), colorectal cancer and gastric cancer. Lenvatinib (LVN), as a multiple-targeted receptor tyrosine kinase inhibitor,1–3 has been approved for several cancers by the American Food and Drug Administration, including HCC,4 thyroid cancer5 and metastatic renal cell carcinoma.6 In a Phase 2 randomized, open, multicenter trial,6 LVN was administered orally, alone or with everolimus, for treatment of progressive metastatic renal cell carcinoma as second-line treatment. The overall survival was not significantly different, LVN alone or combined with everolimus groups had a higher objective response rate and longer progression-free survival. Besides, LVN also showed clinical effect for refractory thyroid cancer. In a Phase 3 randomized, double-blind, multicenter trial, LVN treatment group demonstrated 18.3 months of median progression-free survival, while the placebo group only had 3.6 months of median progression-free survival for patients with radioiodine refractory thyroid cancer. LVN treatment group also had a higher objective response rate (64.8% versus 1.5%).5 In the RELECT study, LVN was shown to be non-inferior to sorafenib with similar survival after treating advanced unresectable HCC patients, thus has been recommended as an optional first-line treatment for unresectable advanced HCC.4 The median survival for LVN treatment group was 13·6 months, which was similar to the sorafenib group with 12.3 months. As such, LVN alone has superior antitumor efficacy in clinical practice with greater potential for combination therapy. The combined LVN and immunopharmacology pembrolizumab treatment showed potential antitumor activity, causing significant survival benefits compared to LVN alone among other cancers.7–10 However, the survival benefits from LVN remain minimal, and treatment-related side effects for these tumors are non-negligible. In this case, an optimal drug delivery strategy for LVN to achieve more excellent therapeutic effects is fundamental. The ideal drug delivery system should have the following features: long-time circulation in vivo, accurate active target in tumor tissue, enhanced synergistic interaction with other therapy means, reduced drug toxicity, etc. Growing studies on LVN drug delivery systems have recently emerged, demonstrating tremendous potential in antitumor role and reducing treat-related side effects. This review assesses and summarizes the current advance of the LVN drug delivery system with an emphasis on how to extend treatment time, improve treatment effect, boost therapeutic effect by combined treatment, and reduce drug toxicity of LVN via nanotechnology.
The Clinical Pharmacological Character of LVN and Antitumor Mechanism
Clinical Pharmacokinetic Parameter of LVN
LVN is approved for oral use in single doses ranging from 8mg to 24mg per day. The specific dosage was determined by the tumor types4–6 and patient weight.11 LVN is primarily metabolized in the liver through CYP3A4.12 LVN is rapidly absorbed after a single dose and the time to maximum concentration (tmax) typically varies from 1h to 4h in healthy volunteers or patients.13,14 However, the pharmacokinetic parameter of LVN is dose-dependent and linear. After once-daily doses, there is no drug accumulation and the relative bioavailability is 90%.15,16 Decyclopropylation, demethylation, N-oxidation, and O-de-arylation are the major principal metabolites of LVN identified from human liver microsomes.12 LVN is primarily excreted via the biliary route in the feces.17 The pharmacokinetic profile of LVN is influenced by drug–drug interactions or disease–drug interactions. However, LVN is unaffected by pH-elevating agents, race and renal function.18 High-fat diet,19 aging,20 impaired liver function and hepatoprotective drugs21,22 could also influence steady-state blood concentrations of orally administered LVN, as shown in Figure 1.
![]() |
Figure 1 Overview of LVN pharmacodynamics and pharmacokinetics in vivo and the time line of indication approval. The target of LVN contains FGFR1-4, VEGFR1-3, c-KIT and rearranged during transfection (RET).The indication of LVN contains for RCC, DTC and HCC. The combination of LVN plus pembrolizumab for RCC and EC as the first-line treatment regimen of adult patients. As the substrate of ATP-binding ABC transporters, P-glycoprotein and BCRP, the maximum concentration of LVN was floated between 1 h-4 h, terminal half-life was about 28 h, apparent oral clearance was ranged from 4.2 L/h to 7.1L/h and terminal volume of distribution from 50.5 L to 163.0 L. Reprinted from Bo W, Chen Y. Lenvatinib resistance mechanism and potential ways to conquer. Front Pharmacol. 2023;14:1153991. Creative Commons.18 |
Regardless of other elements determining the pharmacokinetics of LVN, the actual plasma concentration is the most valuable indicator for patients, and it cannot be replaced by other indirect indicators.18 However, the relative information is limited and inconsistent. Data from the patients with unresectable HCC revealed that the mean trough plasma concentration was approximately 30–40 ng/mL, regardless of the dose of LVN.4 Another study revealed that maintaining concentration above 42.68 ng/mL, identified by the receiver operating characteristic (ROC) curve, is important to achieve objective response rate in patients with HCC;23 however, the study only included 21 HCC patients. However, a study including thyroid cancer patients showed that high plasma levels of LVN appear to be unnecessary for achieving better objective response rate.24 Nevertheless, these individuals experienced increased anorexia and severe hypertension. There is more information available for adverse events reports. According to Nagahama’s study,25 the grades of adverse events, including hypertension, proteinuria, hand-foot syndrome and diarrhea, were not significant different among different trough plasma concentration. Thrombocytopenia is a common adverse sides of LVN, another study found that a decrease in platelet count was correlated with the maximum plasma concentration.26
The Antitumor Mechanism of LVN
Multiple-targeted antitumor mechanisms determine the antitumor efficacy of LVN. LVN is a multiple-targeted receptor tyrosine kinase inhibitor of vascular endothelial growth factor receptors (VEGFR) 1, 2, and 3,1,3 fibroblast growth factor receptors (FGFR) 1 through 4,27 platelet-derived growth factor receptor (PDGFR) α, rearranged during transfection (RET) and stem cell factor receptor (c-KIT) as shown in Figure 1.2,4 It binds to these receptors, inhibits PLCγ, Ras-Raf-ERK, and PI3K-AKT signal pathways, and ultimately influences tumor growth. LVN also suppresses tumor angiogenesis, which causes tumor ischemia and tumor microenvironment hypoxia, resulting in tumor growth suppression and tumor cell death. LVN can block RET phosphorylation and limit the protooncogene c-KIT to reduce cell proliferation.28 Furthermore, LVN can increase CD8+ T cell activity and reduce the number of myeloid-derived suppressor cells (MDSCs), thus also regulating the immune microenvironment.18,28–30 To obtain the most out of LVN, these antitumor mechanisms are adequately used and strengthened by combination therapy.
Resistance of LVN
Since the use of targeted medications in treating malignant tumors has become more common, the problem of drug resistance has increasingly emerged. Cancer treatment with molecularly targeted drugs seems to inevitably be accompanied by primary or acquisition of resistance to the treatment drugs. However, primary drug resistance is gradually declining with the advancement of targeted drugs toward precision medicine based on gene detection. In the REFLECT study, only 15% of patients developed primary drug resistance to LVN, which was much lower than sorafenib of 27%.31,32 A preexisting stable genetic/genetic drug-resistant alteration before therapy attributes to the primary drug resistance.33 Nevertheless, an estimated 85% of patients in the REFLECT study can benefit from LVN, ultimately developing acquired drug resistance 8.9 months after LVN administration.4 Pharmacokinetic parameters changes and molecular targets changes during drug treatment maybe are the main mechanisms of acquired drug resistance, which influence drug efficacy.34 The remodeling of the tumor microenvironment (TME), mutation acquisition, activation of adaptive drug resistance pathways, and stimulation of transporter protein expression are main factors responsible for acquired resistance.34 New therapeutic methods are urgently needed to optimize the pharmacokinetics of LVN, overcome suppressive tumor microenvironments, and switch associated signaling pathways.
LVN-Related Side Effects
LVN often causes early and severe treatment-related side effects. Table 1 shows common treatment-related side effects for LVN in II/III trials. In the REFLECT study, total treatment-related side effects were as high as 94%, Grade 3 side effects related to treatment were up to 75%, and the fatal side effects occurred in 2%, including three patients with liver failure, three patients with cerebral hemorrhage and two patients with respiratory failure. The most common treatment related adverse events were hypertension (42%), diarrhea (39%), anorexia (34%) and weight loss (31%) in sequence.4 A study on renal cell carcinoma patients revealed a high total treatment-related side effects of 94%, and serious adverse events (grades 3 and 4) occurred in 79% of patients with LVN. Proteinuria occurred in 19% and was the most common serious adverse event. Two deaths occurred, including one cerebral hemorrhage and one myocardial infarction.6 Fatigue, appetite loss, hand-foot skin reaction, and diarrhea occurred about one month after LVN administration, whereas hypertension can occur as early as the 4th day.4 Side effects, particularly severe side effects, often result in adverse outcomes, including drug withdrawal, worse health-related quality of life, and short overall survival. In the REFLECT study, side effects causing LVN interruption in 190 (39.9%) patients, dose reduction in 176 (37.0%) patients, and drug withdrawal occurred in 42 (8.8%) patients. The most prevalent adverse events in the study causing dose reductions mainly included hypertension, proteinuria, diarrhea, and fatigue.35 Therefore, strategies for reducing LVN-associated adverse events should be discussed for continued treatment and improvement of survival among patients receiving LVN therapy.
![]() |
Table 1 Treatment-Related Adverse Events Reported in the II/III RCTs |
![]() |
Table 2 The Characteristics of LVN-NDDS |
Therefore, the mechanisms of optimizing LVN pharmacokinetics, overcoming drug resistance, reducing the toxic side effects, and improving antitumor effects are challenges clinicians have to contend with. Nanotechnology is booming, and it has the potential to solve the above problems by actively targeting tumor cells, promoting LVN sensitization, controlling harmful tumor microenvironments, overcoming drug resistance, and reducing toxicity.18 Nano-drug delivery systems (NDDS) have the physicochemical properties of small dimensions, large surface area, and flexible structure, which could make them have the potential as drug carriers.43
Because of their unique features, NDDS can increase drug stability, optimize body distribution, prolong circulation time, as well as improve drug targeting to increase efficacy and reduce possible side effects from the free drugs.43,57–62 Similar to free drugs, the NDDS must also pass through numerous physiological and biological barriers to reach its functional destination. Nevertheless, these NDDS typically possess fundamental features including (1) ability to remain stable in the circulation system until they reach their targets or TME; (2) capacity to escape the reticuloendothelial system (RES) clearance,63 and mononuclear phagocyte system (MPS); (3) efficient accumulation in TME via tumor vasculature; (4) high-pressure penetration into the tumor fluid; (5) reaching the target and only interacting with tumor cells; (6) synergistic effect with combined therapy.64–69
Generations of LVN Based NDDS
NDDS are widely manufactured materials with nanoscale sizes ranging from 1 to 200 nm in at least one dimension,33,70,71 providing innovative therapeutic solutions in medicine, particularly for cancer therapy. NDDS comprises the surface layer, shell layer, and core, which is primarily the main section of the system with the drugs. NDDS has a small diameter and large surface area, which helps improve drug pharmacokinetics and biodistribution.
Its small size enables the NDDS to bypass the biological barriers, hence allowing deep tissue penetration and drug accumulation in multiple organs. Additionally, NDDS has various benefits including tunable thermal, magnetic, targeted, optical, and electrical properties based on different nanomaterials.59–62,65,67–69,72,73 NDDS can also be utilized as a vehicle to co-load multiple drugs, contributing to combination drug therapy to overcome drug resistance and improve treatment effectiveness. Overall, NDDS can improve drug bioavailability, extend drug efficacy, strengthen targeting capacity, and reduce side effects. It is consistently being used clinically with enormous potential for therapeutic benefit. The common NDDS included polymer NDDS, lipid NDDS, silicon NDDS, metal nanoparticles, and biomimetic targeted NDDS.74–76 Recent multifunctional NDDS with numerous functional materials, synergetic drugs, and therapeutic schedules have gained increasing attention because they may provide active devices with better biocompatibility, higher anti-tumor efficiency, and a more widely applications. The NDDS not only significantly improves anticancer properties but also promotes noninvasive tumor diagnosis and monitoring in vivo.77 Several generations of LVN-based NDDS have emerged based on their development and function as exhibited in Figure 2.
![]() |
Figure 2 Generations of LVN-based nano-drug delivery system.The four stages was passive, active, triggered and biomimetic target. |
Passive Target Strategy for LVN
LVN targets specific molecules highly expressed by tumor cells; however, targeted drugs are not usually delivered exclusively into the tumor tissues and are indiscriminately distributed to both tumor and normal tissues with similar molecules target. There is a need to improve NDDS deposition in tumor tissue to increase treatment efficacy and reduce side effects. Nanoparticle transport into tumor tissue include passive transport and active transport. Passive targeting majorly involved in the enhanced penetration and retention (EPR) effect.78 Their nanosized property enables accumulation in tumor sites.79
Unlike normal tissues, the blood vessels endothelium of tumor tissues is more permeable due to their large pores ranging from 200 to 2000 nm.80 This ensures a wider range of small particles entering the tumor tissues only by passive transport, whereas smaller molecule drugs are washed out.77,79,81,82 The misfunction of lymphatic drainage in tumors further enables NDDS to remain inside the tumor longer. Nevertheless, the EPR effect of NDDS can been influenced by several other factors and passive targeting has extremely low efficiency (less than 5%).81,83,84 For instance, studies have shown that advanced large tumors show a weak EPR effect because large tumor can obstruct blood flow and vasculature.81,85,86 In this regard, improving the targeting capacity could bring more gains to the research of NDDS based on LVN.
Active Target Strategy for LVN
To further improve the targeting capacity of NDDS in tumor cells, there is a need for active targeting nanotechnology, ie, adding targeting ligands to the surface of the LVN-nanocarriers to ensure the NDDS selectively bind to specific receptors on tumor tissues.79,87 After ligand recognition with a binding site on the tumor tissues, ligand–target interaction causes in-fold of the tumor cell membrane and internalization of NDDS by receptor-mediated endocytosis.79,88 The common overexpressed specific receptors in HCC with LVN treatment include epidermal growth factor receptor,89 transferrin (40% overexpression rate), lectins, glypican-3 (GPC3),90 folate receptor (approximately 40%)91 and epithelial cell adhesion molecule (EpCAM).92–94 Active targeting is a therapeutic strategy that effectively controls biodistribution and maximizes the accumulation of agents at the tumor cells with minimum toxicity, causing improved efficiency and patient compliance.71,95 In addition to active targeting to cell or cellular organelle, the transcytosable strategy is aimed at targeting to transcytosis for crossing vasculature.96 One of the indication of LVN was HCC, which is highly related to vasculature and angiogenesis. Until now, the strategy was missing in modifying LVN to target to blood vessel. Herein, we believe the transcytosable nanomedicine for LVN would be a very innovative discovery in the near future.
Controllable Drug Release Method for LVN
The above two-generation NDDS majorly adopt diffusion and/or hydrolysis-controlled released systems to release encapsulated LVN outside the target tissue, hence leaving side effects and limiting the efficiency of NDDS.97 Thus, a large dose of LVN could be released into healthy tissues in the absence of a second release control system. Triggered nanocarrier systems have been engineered as the second release control system to release LVN by physiological or pathological status or external stimulus. The stimulus includes internal and external triggers. The internal triggers primarily exist in the tumor microenvironment, including specific enzymes, temperature, acid environment, etc.98–100 For example, the tumor microenvironment always accompanied by high metabolic can generate higher local temperatures (3°C higher) and lower PH, promoting thermos-responsive and pH-responsive NDDS to release LVN into the tumor tissue.74,101 Nonetheless, most of the internal triggers are hardly unique to diseased tissues and thus have low efficiency. External triggers including ultrasound, magnetic fields, electromagnetic radiation and hyperthermia therapy,102,103 photothermal therapy (PTT), and photodynamic therapy are usually added to stimulate specific responses and provide additional physico-therapeutics and biochemical therapy (PDT).88
Biomimetic Tactics for LVN
Another hurdle that must be resolved is that most NDDS have high immunogenicity and can be easily recognized and cleared by the immune system. Biomimetic drug delivery systems, modified by natural or engineered biological cell membrane materials including normal cell membranes or cancer cell membranes, have lower immunogenicity, increased biological active targeting capacity, and better biocompatibility.75,104,105 Cancer cell membrane-camouflaged NDDS with LVN can escape from immunological surveillance and accurately target homoplastic tumor tissues due to membrane proteins expressed by cancer cells.105–107
Until now, the NDDS of LVN has not been registered in the clinical trials. In fact, there are not many clinical applications of nanomedicines. The adriamycin liposomes, daunorubicin B liposomes, paclitaxel albumin nanoparticles are seemed as the representative nanomedicines for antitumor therapy. The limitation for NDDS of LVN could be drawn as these reasons: i) Common problems faced by NDDS are the controllability of scale-up of nanomedicine production and the complexity of the interaction between nanomedicine and complex in vivo environment. ii) Particularity of NDDS of LVN. The adverse effects of LVN in clinic are within acceptable and controllable limits when compared with paclitaxel and adriamycin. The anaphylactic shock caused by paclitaxel and cardiotoxicity caused by doxorubicin could interrupt treatment. The adverse effects caused by LVN are much fewer and milder. Further, the therapeutic activity of LVN is remarkable. After the emergence of LVN resistance, the impact could be made up by replacing second-line treatment drugs. Therefore, the urgency for NDDS of LVN research is not as significant as that of first-line chemotherapy drugs.
Application of NDDS in LVN
The disadvantages of free LVN include rapid elimination rates, limited efficiency, drug resistance, and systemic toxicity. NDDS is rapidly applied to LVN to increase antitumor effects and reduce drug toxicity. The related research efforts for NDDS focus on (1) long-efficiency for overcoming drug elimination; (2) high-efficiency for treatment requirement; (3) synergy-efficiency with other methods for treatment sensibilization and overcoming resistance; (4) tumor tracking and diagnosis; (5) low-toxicity to ensure security as displayed in Figure 3 and Table 2.
![]() |
Figure 3 LVN-NDDS achieved the goals of long-term antitumor effects, higher antitumor effects and diagnosis function. (A) In vitro release curve of LVN at pH=7.4 and at pH=5.3 showed that LVN-NDDS had the characteristic of slow-release; (B) Inhibitory effects of LVN-NDDS on HUH-7 cells and Hep3B cells were stronger that free LVN; (C) MRI test results following coculturing of GPC3/EpCAM-LVN-MLs and HUH-7 cells for 2 and 5 days showed more obvious MRI signals than free LVN. Reprinted from Huang ZL, Li F, Zhang JT, et al. Research on the Construction of Bispecific-Targeted Sustained-Release Drug-Delivery Microspheres and Their Function in Treatment of Hepatocellular Carcinoma. ACS Omega. 2022;7(25):22003-22014. Copyright © 2022 The Authors. Published by American Chemical Society.108 (D) The primary tumor growth and the contralateral subcutaneous tumor growth experiment of Bal b/c mice. The results of the tumorigenesis experiment showed that the tumor growth was slower in the groups treated with LVN-NDDS combined with radiotherapy. Reprinted from Liu J, Chen J, Liu H et al. Bi/Se-Based Nanotherapeutics Sensitize CT Image-Guided Stereotactic Body Radiotherapy through Reprogramming the Microenvironment of Hepatocellular Carcinoma. ACS Appl Mater Interfaces. 2021;13(36):42473–42485. Copyright © 2021 American Chemical Society.46 |
According to Table 2, we realized that HCC was the most studied disease model. The therapeutic aims not only focusing on traditional active or passive target to treat, but also on tracking the specific location of LVN. For lung cancer, early diagnosis was a very crucial link in clinical treatment. The LVN-NDDS was as the targeted nanoparticle contrast agent combined with contrast-enhanced computerized tomography to diagnose early-stage NSCLC. For thyroid cancer, breast cancer and intrahepatic cholangiocarcinoma, the goal for design LNV-NDDS was for target therapy. Next, we would discuss the specific application of LNV-NDDS in different design aims. The methods of NDDS promote the efficacy of LVN are illustrated in Figure 4.
Improving Longer-Term Efficiency of LVN
The capacity of gradual release and stability of LVN should be strengthened for a longer-term anticancer effect. The aqueous solubility of LVN is poor (0.7 μg/mL at 37 ± 0.1 °C), and with low oral bioavailability (about 36%). Thus, a large dosage of LVN is necessary to ensure an effective therapeutic effect, consequently amplifying the extent of drug toxicity.37,110,111 Nanotechnology is a first-rate novel technique to address this limitation. According to Zhang,37 a self-assembled phospholipid and sodium glycocholate oral mixed micelles nano-sized delivery systems sharply improve LVN solubility, slow-releasing ability, bioavailability, and anticancer activity. As integral components of cell membrane, phospholipids possess good biocompatibility and biosafety; sodium glycocholate is used to improve the stability and permeability of the NDDS. With a concentration of 0.35 mg/mL, the micelles significantly increased LVN solubility by 500 times compared to free LVN. This nano-system could also preserve visual stability for at least 1 month at 4°C and the release rate of LVN from mixed micelles is only about 10% of free LVN within 2 hours in vitro. The serum proteins were barely attached to the surface of micelles, indicating good blood compatibility; besides, the hemolytic rate was below 0.5% when the micelles concentration was ≤1 mg/mL. Compared to free LVN, the relative bioavailability of LVN-micelles had been significantly improved by 76.7%. Yoshida44 developed a LVN-incorporating sheet as a controlled release drug delivery system (nanofiber sheets) using poly(ε-caprolactone), a releasing slowly polymer with a constant rate over more than 1 year and approved by the Food and Drug Administration for various biomaterial applications.112 In this study, the nanofiber sheet was designed to continuously slowly release LVN for more than 8 weeks. The release rate was 14.85 ± 0.86% for 2 weeks, 19.15 ± 0.73% for 4 weeks, and 28.02 ± 2.15% for 8 weeks, respectively. In a subcutaneous tumor model, nanofiber sheets had a sustained-release effect with serum level peaked at 24h and a gradual downward trend until day 14; the free LVN group had the serum level peaked at 3h but disappeared at 24 h. The nanofiber sheets contained less LVN (32.5% of the free LVN group), but the nanofiber sheets showed better tumor inhibition capacity, better 30-day survival in the HCC xenograft model, and lower toxic and side effects.
Achieving Higher Efficiency of LVN
For higher efficiency of LVN, an active targeting strategy is applied for selective delivery of more LVN to the tumor tissue to exert strong pharmacological activity. FR, a transmembrane protein, is usually overexpressed on the surface of HCC cells113 and intrahepatic cholangiocarcinoma (ICC) cells.114 Ning39 developed a targeting nano-delivery system using hollow manganese dioxide materials modified by folate to actively target 9810 ICC cells. The NDDS could be easily taken in by tumor cells and sustain LVN release. After 48h administration, the NDDS caused cell viability decreasing at least 60% which is significantly 1.5 times higher than the free LVN administration (40%). Herein, folate modification could improve the intracellular delivery of LVN and the efficacy of the anticancer properties of LVN. Additional studies have shown that the NDDS system could significantly improve the pro-apoptosis effect of LVN by repressing Raf1-MEK1/2-ERK1/2 signaling path.
TME has peculiar features from normal tissue and is key for tumor cell growing. It can also promote immunosuppression and drug resistance, thus promoting tumor metastasis.115–120 The corresponding TME influences the effect of NDDS; however, nanotechnology has been developed to overcome the adverse factor from the TME and has been explored for reprogramming the tumor microenvironment.100,121 The TME mainly includes three parts, ie, cellular components (all the non-cancerous host cells in the tumor, including fibroblasts,122 endothelial cells,123 and infiltrating immune cells), non-cellular components and non-cellular components of the TME (for example, an acidic environment, hypoxic conditions, and extracellular matrix).100,121
Notably, TME remains an obstacle to achieving high efficiency for LVN; nonetheless, the unique environment can also be exploited to improve the targeting of the NDDS. An important feature in the tumor intracellular conditions is a high glutathione (GSH) concentration, ie, 50–1000 times higher than that in extracellular microenvironments.124 GSH concentration differences have been used to construct targeted drug delivery and release nanoparticles.125–127 Ding47 developed a nanogel system loading LVN in the hydrophobic core (NG/LVN); the study results showed that the cumulative released amount of LVN from nanogel was 91.6% in the mimic enriched GSH microenvironments, but only 65.4% when no GSH was present. This NG/LVN system was developed as a GSH-responsive drug release system and its antitumor effect was observed in HCC tumor-allografted mice. After treatment, the smallest tumor weight in the NG/LVN group was 0.15 g, which was barely half of the tumor weight of 0.34g in the free LVN group. The excellent anti-tumor ability of NG/LVN was attributed to inducing large area tumor necrosis in the tumor tissue.
However, targeting roles of single surface molecules on NDDS have imprecise selectivity, poor binding power, and insufficient blood circulation time. A combination of multiple molecules or TME-responsive characteristics is key to further optimizing these functions. Ferritins can be artificially expressed under iron-free conditions, leaving enough space that can be loaded with different drug, and can be selected as an ideal drug delivery system with biocompatible and homogeneous characteristics.128,129 Additionally, ferritins can selectively target cancer tissues through binding receptors commonly expressed in cancer cells.130 Skubalova53 constructed an NDDS using ferritins and surface-functionalized with folic acid. These double-targeted NDDS were encapsulated with LVN and vandetanib to exert significantly higher anticancer activity.
Cell membrane camouflage technology has been widely employed to manufacture nanodrugs with improved targeting because it allows immune escape and improves blood circulation time. Wu created an NP system with a core of LVN wrapped in a pH-sensitive polymer, and a shell made of a cancer cell membrane derived from SMMC 7721 cells.43 Inherent homologous adhesion properties (EpCAM, N-cadherin, galectin-3, and CD-147) on the cancer cell membrane could determine an adhesive connection between NDDS and tumor tissue and promote NDDS aggregation around tumors. This NDDS is a pH-responsive copolymer which can self-assemble into nanoparticles in a neutral environment and can cause the decomposition of NDDS in the TME acidic environment. With an excellent enrichment in tumor and a high release rate of 67.1% after 96 hours, this system effectively caused LVN enrichment in tumor tissues and markedly inhibited tumor growth even on the 21st day. The NDDS caused approximately 81% cell death in vitro, which was two times higher than the free LVN (34%). Additionally, the NDDS system induced a 63.18% apoptosis rate, which was almost two times higher than free LVN (33.27%).
Although the data of LVN-NDDS in experiment were positive, the feasibility in clinical applications still faced with some obstacles. i) Liposome and nanoparticle are the only formulations of NDDS in clinic. Herein, the optional materials for NDDS are restricted. If the LVN-NDDS needs to be marketed in the future, it needs to be selected among nanomaterials that have been evaluated for marketing safety. ii) The parameters of entrapment efficiency, drug loading and drug release rate of LVN-NDDS are faced with challenges as exhibited in Table 2. The lowest entrapment efficiency, drug loading rate were 21% and 2%, respectively. The lower entrapment efficiency and drug loading rate means the lower LVN in NDDS, which would greatly limit the need for drug dosage in clinical use. iii) The third difficulty for LVN-NDDS would be concluded by the rate of drug release. As in Table 2, some LVN-NDDS could not be fully released. The drug release could not reach half of the drug.
Herein, a more suitable formulation process upgrading is necessary.
Combined Strategy of LVN
Since the use and efficacy of NDDS with single drug are extremely limited, combining them with other emerging therapies is hopeful and becoming a hot topic. Numerous efforts have been made to combine therapy, providing promising synergistic therapeutic benefits and tolerable safety. Notably, chemotherapy, immunotherapy, radiotherapy, and PDT are the most common combination therapies.
Combination Chemotherapy Therapy and LVN
Adriamycin is an anthracycline antibiotic that has been used for treating several cancer types,131 including HCC. Besides, adriamycin can induce tumor cells immunogenic cell death109 and subsequently trigger additional cellular immune responses.132 Zhang40 co-assembled a metallo-nanodrug (Fe-LVN/Adr@EGCG) of LVN, adriamycin, Fe3+ ion, and a natural polymer. The fluorescence results revealed that nanodrugs strongly accumulated in the liver tumor tissues compared to free LVN. The acid TME (pH 6.5) can act as a stimulus to trigger NDDS and regulate drug releasing into tumors, which was 1.8 folds higher than that in pH 7.4. The nanodrugs exhibited excellent antitumor effect and substantially slowed tumor growth, with 3/6 tumor complete remission on the 42nd day in mice. The survival rate on day 42 was 66.6%, which was fourfold higher than free adriamycin (16.6%) and twofold higher than LVN (33.3%) treated groups. The findings revealed that cell viability of nanodrugs treated patient-derived organoids sharply decreased to 35.5%, which was at least two folds lower than that of free LVN (100.3%) or adriamycin (105.7%) treated patient-derived organoids from the same patient. These metallo-nanodrugs also displayed their multifunction role, including efficient drug accumulation, slow-release, promotion of tumor vascular normalization, and immunological enhancement.
Combination of Traditional Chinese Medicine Therapy and LVN
Many previous studies have showed that combinations of Chinese medicine and chemotherapeutic agents increase efficiency and decrease toxicity.133–135 Bufalin, deriving from the traditional Chinese medicine Chansu, exerts antitumor capacity by decreasing cancer cell proliferation and inducing cell apoptosis, necroptosis, autophagy, and senescence.136–138 Studies indicate that the combination of bufalin and sorafenib yields a synergistic antitumor effect.139 Ning38 designed a co-delivery nano-system with LVN and bufalin. Monodisperse mesoporous silica nanoparticle was synthesized and sequentially modified by folic acid. This system improves the targeting property, internalization rate, and slow-release capacity. Consequently, this system treatment not only significantly suppressed the 9810 cells’ viability, migration, and invasion, but also more remarkably inhibited tumor growth, unlike single LVN or bufalin in orthotopic xenograft nude mice model of ICC. Moreover, the NDDS provides a useful method to highly target tumor cell and reversal multidrug-resistance for ICC.
Combination Radiotherapy and LVN
Radiotherapy is an important treatment option for many cancers and many innovative radiotherapy techniques have recently been applied to liver cancer.140,141 Stereotactic body radiotherapy is a modern radiotherapeutic modality that precisely targets tumors and delivers high radiation doses; it has been shown to exert equivalent effects on surgery in early-stage HCC.142 However, the tumor microenvironment with hypoxia and immune suppression, and inaccurate imaging guidance inhibit radiation-induced anticancer efficacy.143 Liu46 developed a novel and compound NDDS to reverse hypoxia and alter the immune suppressive microenvironment, allowing image-guided HCC radiotherapy to be realized. LVN was loaded to this NDDS system to promote tumor vascular remodeling, ameliorating hypoxic state and improving immune cell recruitment and aggregation, including tumor infiltrating CD8+ T lymphocyte, natural killer (NK) cell, and CD4+ T lymphocyte. Bi quantum dots were loaded to serve as a computerized tomography (CT) contrast agent for achieving the image-guided radiotherapy. Se was used to protect Bi from oxidation. The Bi/Se-LVN NDDS system combined with radiotherapy showed the most excellent radiosensitization capacity in vitro with a sensitization enhancement ratio ranging from 2.36 to 3.12, which was nearly two times the Bi/Se NDDS group (1.21–1.83) and free LVN group (1.45–1.47). Taken together, NDDS combined with radiotherapy showed strong antitumor capacity by promoting cell apoptosis through the Compton and photoelectric effects. Bi/Se-LVN NDDS could improve CT imaging in vivo and further get a more accurate alignment of the radiotherapy target area with the most excellent antitumor effect. The combination of LVN with technical elements related to radiotherapy not only could enhance the effect of LVN but also supply the new way for radiotherapy with chemical drug in clinic. The radiotherapy could reach inside the tumor cells and destroy them. At this time, small molecule targeting drugs directly into the tumor cells, giving the tumor cells a second blow, can curb tumor recurrence and metastasis. This strategy could be an entirely new way to deliver small molecule targeted drugs in the future.
Combination of PTT and LVN
Hyperthermia treatment is a fast-expanding antitumor method that is making advances in cancer therapy.144 When temperatures reaches 41°C, hyperthermia can cause vessel dilation and increase blood perfusion,145 resulting in a heat shock response in tumor cells and subsequently immune activation.146 Irreversible cell damage and acute coagulative necrosis occur at temperatures of 46°C.147 Conventional hyperthermia treatment increases the tumor temperature in an outside-in method, therefore causing dissipation of most thermal energy and bringing extra injury to surrounding healthy tissues. PTT uses NDDS embedded within tumors as exogenous energy absorbers to convert light energy into heat energy, causing irreversible tumor cellular injury and subsequent tumor regression. This treatment strategy could increase the temperature in an inside-out way; the tumor tissue will heat up from its internal parenchyma, leaving the surrounding normal tissues mostly uninjured.148 This functional NDDS show a potential treatment strategy for numerous cancers.149–151 Various nano-materials including polydopamine (PDA), poly lactic-co-glycolic acid (PLGA), gold nano-material, and superparamagnetic iron oxide nanoparticles have been used as PTT agents for antitumor treatment. On account of the limited tissue penetration capacity of light, PTT is more appropriate for superficial tumors than deep tissue tumors, eg thyroid and breast cancers.152 PTT has strong absorption in near-infrared (NIR) regions, excellent photothermal conversion efficiency, fantastic biocompatibility and biodegradability. As a non-invasive antitumor method, PTT is showing more promising antitumor effect than conventional cancer therapy, especially when PTT combines with LVN.
Giammona48 designed a NIR-activated NDDS system using gold nanorod nanocomposites coated with LVN. This system, decorated with galactose residues, is designed to promote the internalization of nano-systems in tumors by targeting the asialoglycoprotein receptor, which is abundantly expressed by hepatocytes. The LVN release induced by the NIR irradiation was about as 2.5 times as the non-irradiated nano-systems. After laser treatment, empty NDDS and LVN-NDDS did not, however, reveal any significant cytotoxic difference on tumor cell lines, suggesting that cell death was caused predominantly by the PTT effect, rather than LVN. Some studies have proved that PTT has a synergistic enhancement antitumor effect with LVN. Zhou36 fabricated multifunctional lanthanide-doped upconversion nanoparticles (called UCNP@PDA@LVN) by loading LVN for treating anaplastic thyroid cancer. The NDDS were irradiated by the 808 nm NIR-laser for 10 minutes; the temperatures of tumor cells increased to 55 °C. Tumor cells can be efficiently damaged when temperatures increase above 50 °C for several minutes. The NDDS had an excellent photothermal conversion rate of 30.7%, significant photothermal conversion stability, and reproducibility with rapid heating and cooling patterns. Additionally, PTT can effectively stimulate LVN release accumulating it at the tumor site. The 24-hour release rate of LVN was about 22%, but it increased to 34% after NIR-laser irradiation, with lower cytotoxicity than free LVN. The viability of C643 cells was 32% when the NDDS was administrated; however, they significantly decreased to 13% upon irradiation by NIR-laser for 10 min. In C643 tumor-bearing mice, the NDDS combined with PTT strongly inhibited tumor growth with a nearly complete suppression over 14 days, unlike the NDDS-alone group.
In using PTT treating deep tumors including HCC, Xu49 used the NIR-II zone as energy for irradiation. The multifunctional NDDS system includes PLGA as a nanocapsid, coated with LVN and copper sulfide nanocrystals (Cu2-xS NCs) which has excellent photothermal properties in the NIR-II. As the rate of hydrolysis of PLGA changes with temperature and pH;153 the release rate of LVN also changes corresponding. Approximately 88.7% of LVN was released within 72 hours from this platform when a laser and an acidic environment happened, which was nearly 1.5 times higher than that without laser irradiation (62.5%). Cu2+ was confirmed to catalyze H2O2 decomposition in vitro to generate oxygen and remarkably increase oxygen concentration in TME. Unlike free LVN, this nano-system could also significantly reduce GSH level to 57.1%, which was confirmed to be closely related to MDR by promoting P-gp expression. The thermal imaging camera monitor showed that this NDDS has good photothermal stability with only small fluctuations and could reach a temperature of 56.1°C in 10 min, with a photothermal conversion rate of 31.07%. With NIR-II laser irradiation, cell viability decreased from 82% to 29%. With a near-infrared laser, the NDDS group mice reached temperatures of 54.3 °C; however, the PBS control group only had a temperature of 5.3 °C. With 14-day treatment, the average weight of tumors in the free LVN group and NDDS group were 0.65 g and 0.31 g, but the tumor in the NDDS plus laser group had a weight of only 0.02g. The NDDS plus laser groups showed a 30 times tumor reduction effect than free LVN; pathology found that nearly the entire tumor area was necrosis and the expression of P-gp decreased to 10.6%. The application of PTT significantly increased LVN-mediated tumor inhibition effects.
Combination of PDT and LVN
PDT uses reactive oxygen species (ROS) generated from photosensitizers by specific wavelength laser activation,154,155 causing an apoptotic or necrotic response to tumors.156 The ROS can effectively kill tumor cells and PDT is a promising treatment intervention against cancer. Zong157 established a photosensitizer NDDS combined with LVN. Due to its efficient singlet oxygen yield (the most prominent ROS), the halogenated boron-dipyrromethene was used as photosensitizer and LVN was added to achieve the synergy antitumor effects. To improve the stability, pluronic F127 which had a hydrophilic outer part and a hydrophobic inner part was used to encapsulate the above drugs. The acidic TME stimulated the core-shell structure to degrade and release halogenated boron-dipyrromethene and LVN. The boron-dipyrromethene had low toxicity in the dark; however, under laser irradiation, halogenated boron-dipyrromethene was strongly phototoxic by producing a significant amount of ROS in Hep3B/Huh7 cells. Upon laser irradiation 5 min, the NDDS made 50% of the Hep3B cells killed in vitro, which was 2.5 times higher than that without irradiation (20%). Meanwhile, the NDDS could upregulate apoptosis-related proteins and promote HCC cell apoptosis. Laser irradiation resulted in 47.8% of the Hep3B cell apoptosis, which was much more than those without irradiation (30.4%). The underlying synergistic mechanism confirmed that ROS enhanced the permeability of mitochondrial membranes; therefore, the Bcl-2 could be released into the cytoplasm, inducing tumor cell apoptosis. Wei54 identified another synergistic mechanism of LVN combined with PDT, ie, generated ROS can deplete intracellular GSH levels to downregulate P-gp expression and reverse LVN resistance. Herein, a photosensitive multifunctional conjugate ZnPc-C8-LVN system was carried out to realize PDT. Compared to free LVN, ZnPc-C8-LVN upon irradiation caused at least a 2-fold reduction of the GSH level and P-gp expression reduction in MCF7/ADR cells. ZnPc-C8-LVN system suggested a significantly improved antitumor activity against MCF7/ADR cells, with IC50 values of 47.32 uM, a 6.9-fold decrease compared with free LVN, indicating that ZnPc-C8-LVN system could reverse MDR in tumor cells. This NDDS could also improve cell apoptosis through the Bcl-2/caspase 3 pathway by decreasing GSH and monitoring the changes of NDDS in vivo in real-time using the fluorescence characteristics. Research on PDT and PTT combination has revealed excellent synergistic antitumor effects. Pan52 designed a liposome-based nano-system which was fabricated by simultaneously encapsulating IR 780 as a photosensitizer and photothermal agent, and LVN, together with banoxantrone (AQ4N) molecule as the hypoxia-activated prodrug. Under an 808 nm laser irradiating IR 780, abundant ROS were produced and the oxygen in tumor tissues was rapidly exhausted, resulting in worsen hypoxia. The hypoxia TME made non-toxic AQ4N convert to toxic AQ4, which had excellent anti-tumor toxicity.158,159 The photothermal conversion efficiency was as high as 52%. In mice bearing subcutaneous 4T1 tumors, the nano-system plus laser treatments showed incredible antitumor effects, which caused complete tumor elimination in the treatment endpoints; however, the free LVN group and nano-system alone group had tumor volumes of approximately 600mm3 and 300 mm3, respectively. Furthermore, fluorescence-based imaging derived from NDDS could be used to diagnose metastatic lymph nodes.
Combination Chemodynamic Therapy (CDT) and LVN
CDT is another novel cancer therapeutic strategy without invasiveness that has attracted tremendous attention due to its special advantages.160,161 The therapeutic principle is based on the fact that TME generally overproduces H2O2 (50–100 × 10–6 M). CDT agents can convert H2O2 into the hydroxyl radical at the tumor region, ie, the most harmful ROS by Fenton/Fenton-like reactions, causing c apoptotic cell death.162,163 Its benefits include high tumor-selective, reduced side effects, regulating the hypoxic and immunosuppressive TME as well as low cost.164 CDT has been used to combine with other treatments, including chemotherapy and PTT to achieve enhanced anticancer effects. Xu42 developed multimodal synergistic theragnostic nanoplatforms for LVN delivery, with hyperthermia/pH-enhanced Fenton-like catalytic activities and GSH/glucose depletion. In this system, copper sulfide (Cu2-xS) nanocrystals, gold nanoparticles, and LVN were simultaneously encapsulated into the NDDS. Gold nanoparticles had nano-enzyme effects to catalyze Cu2-xS for excellent Fenton-like effects. The system was supplemented by galactosamine to provide biocompatibility and implement an active targeting effect. The synergistic action of Cu+ and gold improved the peroxidase effect to generate hydroxyl radicals, promote Cu2+ consumption of GSH and glucose, and catalyze H2O2 to produce oxygen, increasing oxygen by 2.26 mg/L compared to Cu2+ alone. By the NIR-II irradiation, the temperature can increase from 48 °C to 60 °C. The photothermal conversion efficiency was 39%. Cell viability of MHCC97H cell experiment found that the combination therapy with PTT and CDT showed a more excellent antitumor effect, with a survival rate of 9.3%, which decreases by 10%–20% compared to treatment alone. The NDDS plus 1064 nm laser had superior antitumor efficacy, confirmed by at least 1-fold reduction of tumor weight and typical histopathological damage. Generally, combined PTT and CDT therapy significantly improves the efficacy of LVN and enriches the NDDS-augmented multimodal synergistic HCC therapy modality.
Combination of Immunotherapy and LVN
The immune microenvironment in tumors is immunosuppression and could promote cancer evasion from immunosurveillance.119 The immunosuppressive TME primarily comprises immunosuppressive cells, soluble cytokines, and signaling cascades.165 The tumor-associated immunosuppressive cells, including myeloid-derived suppressor cells, regulatory T Cells, M2 tumor-associated macrophages (TAM) (tumor-promoting subtype), etc., mediate the development and deterioration of tumors and chemotherapy resistance. The immunosuppressive TME is common in tumor tissues with anti-angiogenesis treatment.123 For instance, HCC often develops drug resistance to LVN after an initial good therapeutic response and the immunosuppressive cells gradually infiltrate into the treated tumor site, including the TAMs.166 By secreting growth factors and inflammatory cytokines, TAMs could stimulate angiogenesis and suppress antitumor immunity, causing revascularization and tumor progression.167 Although TAMs have ability of tumor-promoting (M2 phenotype), remodeling macrophage plasticity could switch M2 to a tumor suppression phenotype M1. The reprogramming of suppressive immune and tumor-promoting TME provides a promising cancer therapy strategy. The TLR7/8 agonist enables TLR7/8 activation and macrophage polarization toward M2-type TAMs. To promote the anti-vascular therapy efficiency of LVN, Liu45 constructed a supramolecular hydrogel NDDS co-assembled with LVN and TAMs-reprogramming polyTLR7/8a nano-regulators for liver cancer treatment. LVN played a role of destroying angiogenesis in tumors and reducing microvessel density; polyTLR7/8a nano-regulators repolarized pro-angiogenic M2 subtype into anti-angiogenic M1 subtype. The NDDS promoted 20.8% of M2-type macrophages to switch into the M1 phenotype, whereas that of the PBS group was 2.48%. After 7-day treatment in C57BL/6 mice, the CD31(+) vascular area in the combination group (polyTLR7/8a and LVN) was reduced by 1.19 times that in the LVN delivery system group. In C57BL/6 mice with 14 days of treatment, the combination treatment group reduced tumor burden by 22% and increased necrotic tumor by 39.8% than the LVN delivery system group. These findings suggest that LVN combined with TAMs reprogramming regulator can improve anti-angiogenic treatment effects for orthotopic HCC.
In another study51 focusing on metastatic triple-negative breast cancer, LVN and vadimezan (a stimulator of interferon genes agonist) were wrapped into synthetic high-density lipoprotein NDDS. As a result, LVN killed cancer cells and vadimezan stimulated local inflammation to promote dendritic cell maturation and M1 macrophage differentiation, synergistically increasing the infiltration of total and active cytotoxic T lymphocytes in tumor by 33- and 13-fold, respectively. The antitumor efficacy of this NDDS was assessed on mice with orthotopic 4T1 tumors. After three doses of NDDS treatment, the weight of tumors reduced by 73.4%, the doses were 1.6 times more effective than LVN alone (slowed tumor weight by 44.7%). This highlights the use of LVN combined with immunopotentiator as a promising treatment.
Reducing Toxicity
Studies have shown that NDDS not only improves the therapeutic effect of LVN but also decreases its associated toxicity. The excellent characteristic such as slow release, good targeting, controllable drug release, and reduction in the volume of distribution, LVN NDDS may possess relatively low drug toxicity.168,169 Most studies on NDDS-LVN have shown that LVN encapsulated in NDDS has similar hematology analysis results, liver and renal function compared with free LVN.38,41,46,52 Histopathological examination revealed no organ injury or inflammation in NDDS-LVN treated animals compared to free LVN. Interestingly, one study showed lower cytotoxicity of LVN in NDDS at equal concentrations.36 Another study reported that the aspartate transaminase and lactate dehydrogenase value on day 14 in the LVN-NDDS group were significantly lower than the free LVN oral group.44 The reason might be related with the different pharmacological characters. The serum LVN levels in the oral group peaked at 3 h after administration and disappeared at 24 h, while the LVN-NDDS group peaked at 24 h and then maintained a gradual downward trend. The decreased distribution volume and sustained release profile enhanced the therapeutic effect and decreased the toxicity in vivo. In addition, mild histological injury in the liver and heart was detected in the free LVN-treated mice, but not in the LVN-NDDS group. Further analysis revealed that disordered cardiomyocytes and broken muscle fibers were major pathological changes. In addition, irregular hepatic lobules structure also occurred, and some hepatocytes exhibited mild staining intensity and were slightly vacuolated.47 These findings suggest that LVN-NDDS can reduce LVN toxicity on hematology and histology levels.
In terms of extra toxicity associated with NDDS, most studies found that LVN-NDDS had good biosafety profile and had negligible side effects compared with PBS.36,39,40,43,45–47,49–52,54 However, several studies reported that LVN-NDDS was primarily accumulated and distributed to the liver36,42,43 and spleen42 except for the tumor. Overall, these results demonstrate that LVN-NDDS can effectively kill cancer cells without causing significant toxicity.
Future Perspective
The antitumor effects of free LVN are limited owing to poor targeting ability, slow releasing, poor solubility and permeability.170 The LVN-NDDS given more chance for LVN treatment. Despite the superior advantage of LVN-NDDS, several limitations exist in the field of LVN-NDDS which need to be addressed in the future.
i) The therapeutic efficacy is not consistently uniform, as discussed in the review. Although active targeting seems to be an effective strategy for delivering drugs to tumor sites, the specific molecules highly expressed on tumor cell surface may not be exclusive to tumor cells.95,171,172 For example, the folate receptor is highly overexpressed in ovarian, lung and breast cancers, and is an excellent drug target. However, folate receptor is also expressed in normal tissues of the choroid plexus, thyroid, colon and bladder, which limits its targeting specificity.173 Moreover, single or double targeting molecules may not be recognized by all tumor cells because tumor heterogeneity leads to multiple subtypes.174,175 Therefore, multiple targeting strategy with different types of molecules or homologous cancer cell membrane-camouflaged nanoparticles should be developed in future to improve the therapeutic targeting of the molecules.
ii) The health and safety implications of LVN-NDDS have not been clarified.176 Although many studies have proved the short-term biosafety of NDDS, their potential toxicity should not be ignored given the long-term and abundant accumulation of NDDS in the body. Following oral or intravenous administration, most NDDS are directed to specific target sites through EPR effects or active targeting. However, some NDDS may still be deposited in other organs via systemic blood circulation. This toxicity is mediated by the production of factors such as ROS, proinflammatory cytokines, oxidative stress, phagocytosis impairment, enhanced permeability of barriers, and fibrosis.177 ROS induced by NDDS has been shown to contribute to toxicity of various organs.178,179 Further, Corona effect casused by NDDS could not be ignored.
iii) More biodegradable nanomaterials should be developed to deliver LVN, such as Fe3O4 and PLGA. These materials can be metabolized in vivo, and the material itself can be used as a metabolic substance or treat related diseases.180
iv) The route between LVN and LVN-NDDS was different. The clinical outcome for oral or intravenous injection might bring different outcome in clinic. For LVN-NDDS, there is no corresponding clinic trial. The efficacy and adverse effect would gain more attention, especially when the administration route changed.
v) Future studies should construct LVN-NDDS combination therapy with local therapy, such as transcatheter arterial chemoembolization (TACE) and ablation. TACE and ablation have been demonstrated to be effective treatments for HCC, but usually leaving inadequate tumor necrosis. A randomized, controlled clinical showed that thermosensitive liposomal doxorubicin plus RFA resulted in better tumor necrosis and prolonged the survival of patients with large HCC.181 Another study combined targeted nanoparticle-delivery sorafenib system and TACE also suggested that TACE plus sorafenib NDDS exhibited better disease control rate. As a monotherapy drug, LVN has the best ORR and longest survival compared with other drugs in HCC.182 LVN-NDDS combined with local treatment are potential approaches for HCC treatment in future.
Conclusion
Despite existing challenges, LVN-NDDS have shown promising potential as a tumor therapy regimen that overcomes MDR and enhance antitumor efficacy. Future research should aim to develop even more intelligent and multifunctional LVN-based NDDS for clinical application.
Abbreviations
HCC, hepatocellular carcinoma; LVN, lenvatinib; VEGFR, vascular endothelial growth factor receptors; FGFR, fibroblast growth factor receptors; PDGFR, platelet-derived growth factor receptor; RET, rearranged during transfection; c-KIT, stem cell factor receptor; MDSCs, myeloid-derived suppressor cells; AUC, area under the plasma concentration vs time curve; Cmax, maximum plasma concentration; CYP3A4, cytochrome P450 3A4; ABC, ATP-binding cassette; P-gp, P-glycoprotein; ROC, receiver operating characteristic curve; ORR, objective response rate; TME, tumor microenvironment; NDDS, Nano-drug delivery systems; RES, reticuloendothelial system; MPS, mononuclear phagocyte system; EPR, penetration and retention; EGFR, epidermal growth factor receptor; GPC3, glypican-3; FR, folate receptor; EpCAM, epithelial cell adhesion molecule; PTT, photothermal therapy; PDT, photodynamic therapy; ICC, intrahepatic cholangiocarcinoma; GSH, glutathione; RT, Radiotherapy; NK, natural killer; PDA, polydopamine; PLGA, Poly lactic-co-glycolic acid; NIR, near-infrared; TAM, tumor-associated macrophages; MRI, magnetic resonance imaging; CT, computerized tomography; PET/CT, positron emission tomography-CT.
Acknowledgments
This work was supported by Natural Science Foundation of Chongqing (No. cstc2021jcyj-msxmX0467); Open Fund of Personalized Drug Therapy Key Laboratory of Sichuan Province (No. 2021YB03); Sichuan Cancer Hospital Outstanding Youth Fund (No. YB2023026); Clinical Research Project of Sichuan Anticancer Association (HX2023-Free Exploration 002) and Sichuan Provincial Natural Science Foundation of China (No. 24NSFSC1116).
Author Contributions
All authors made a significant contribution to the work reported, whether that is in the conception, study design, took part in drafting, revising or critically reviewing the article; gave final approval of the version to be published; have agreed on the journal to which the article has been submitted; and agree to be accountable for all aspects of the work.
Disclosure
The authors declare no conflicts of interest in this work.
References
1. Matsui J, Funahashi Y, Uenaka T, Watanabe T, Tsuruoka A, Asada M. Multi-kinase inhibitor E7080 suppresses lymph node and lung metastases of human mammary breast tumor MDA-MB-231 via inhibition of vascular endothelial growth factor-receptor (VEGF-R) 2 and VEGF-R3 kinase. Clin Cancer Res. 2008;14(17):5459–5465. doi:10.1158/1078-0432.CCR-07-5270
2. Matsui J, Yamamoto Y, Funahashi Y, et al. E7080, a novel inhibitor that targets multiple kinases, has potent antitumor activities against stem cell factor producing human small cell lung cancer H146, based on angiogenesis inhibition. Int, J, Cancer. 2008;122(3):664–671. doi:10.1002/ijc.23131
3. Yamamoto Y, Matsui J, Matsushima T, et al. Lenvatinib, an angiogenesis inhibitor targeting VEGFR/FGFR, shows broad antitumor activity in human tumor xenograft models associated with microvessel density and pericyte coverage. Vasc Cell. 2014;6:18. doi:10.1186/2045-824X-6-18
4. Kudo M, Finn RS, Qin S, et al. Lenvatinib versus sorafenib in first-line treatment of patients with unresectable hepatocellular carcinoma: a randomised phase 3 non-inferiority trial. Lancet. 2018;391(10126):1163–1173. doi:10.1016/S0140-6736(18)30207-1
5. Schlumberger M, Tahara M, Wirth LJ, et al. Lenvatinib versus placebo in radioiodine-refractory thyroid cancer. N Engl J Med. 2015;372(7):621–630. doi:10.1056/NEJMoa1406470
6. Motzer RJ, Hutson TE, Glen H, et al. Lenvatinib, everolimus, and the combination in patients with metastatic renal cell carcinoma: a randomised, phase 2, open-label, multicentre trial. Lancet Oncol. 2015;16(15):1473–1482. doi:10.1016/S1470-2045(15)00290-9
7. Choueiri TK, Eto M, Motzer R, et al. Lenvatinib plus pembrolizumab versus sunitinib as first-line treatment of patients with advanced renal cell carcinoma (CLEAR): extended follow-up from the phase 3, randomised, open-label study. Lancet Oncol. 2023;24(3):228–238. doi:10.1016/S1470-2045(23)00049-9
8. Makker V, Colombo N, Casado Herráez A, et al. Lenvatinib plus Pembrolizumab for Advanced Endometrial Cancer. N Engl J Med. 2022;386(5):437–448. doi:10.1056/NEJMoa2108330
9. Mo DC, Luo PH, Huang SX, Wang HL, Huang JF. Safety and efficacy of pembrolizumab plus lenvatinib versus pembrolizumab and lenvatinib monotherapies in cancers: a systematic review. Int Immunopharmacol. 2021;91:107281. doi:10.1016/j.intimp.2020.107281
10. Motzer R, Alekseev B, Rha SY, et al. Lenvatinib plus Pembrolizumab or Everolimus for Advanced Renal Cell Carcinoma. N Engl J Med. 2021;384(14):1289–1300. doi:10.1056/NEJMoa2035716
11. Tamai T, Hayato S, Hojo S, et al. Dose Finding of Lenvatinib in Subjects With Advanced Hepatocellular Carcinoma Based on Population Pharmacokinetic and Exposure-Response Analyses. J Clin Pharmacol. 2017;57(9):1138–1147. doi:10.1002/jcph.917
12. Ikeda M, Okusaka T, Mitsunaga S, et al. Safety and Pharmacokinetics of Lenvatinib in Patients with Advanced Hepatocellular Carcinoma. Clin Cancer Res. 2016;22(6):1385–1394. doi:10.1158/1078-0432.CCR-15-1354
13. Mano Y, Mizuo H. Minimal impact of hepatic and renal impairment on plasma protein binding of lenvatinib, and identification of its major plasma binding protein. Biopharm Drug Dispos. 2019;40(8):307–311. doi:10.1002/bdd.2204
14. Shumaker R, Aluri J, Fan J, Martinez G, Thompson GA, Ren M. Effects of Ketoconazole on the Pharmacokinetics of Lenvatinib (E7080) in Healthy Participants. Clin Pharmacol Drug Dev. 2015;4(2):155–160. doi:10.1002/cpdd.140
15. Catalano M, Casadei-Gardini A, Vannini G, et al. Lenvatinib: established and promising drug for the treatment of advanced hepatocellular carcinoma. Expert Rev Clin Pharmacol. 2021;14(11):1353–1365. doi:10.1080/17512433.2021.1958674
16. Gupta A, Jarzab B, Capdevila J, Shumaker R, Hussein Z. Population pharmacokinetic analysis of lenvatinib in healthy subjects and patients with cancer. Br J Clin Pharmacol. 2016;81(6):1124–1133. doi:10.1111/bcp.12907
17. Shumaker R, Aluri J, Fan J, Martinez G, Ren M, Chen K. Evaluation of the effects of formulation and food on the pharmacokinetics of lenvatinib (E7080) in healthy volunteers. Int J Clin Pharmacol Ther. 2014;52(4):284–291. doi:10.5414/CP201937
18. Bo W, Chen Y. Lenvatinib resistance mechanism and potential ways to conquer. Front Pharmacol. 2023;14:1153991. doi:10.3389/fphar.2023.1153991
19. Wei Y, Yu Y, Zhang W, et al. Effects of diet and gender on the pharmacokinetics of oral lenvatinib: a clinical trial in healthy Chinese participants. Int J Clin Pharmacol Ther. 2023;61(11):475–481. doi:10.5414/CP204440
20. Du X, Cai H, Jin N, et al. Differences in the pharmacokinetics and steady-state blood concentrations of orally administered lenvatinib in adult and juvenile rats. Front Pharmacol. 2023;14:1140849. doi:10.3389/fphar.2023.1140849
21. Cui Y, Li Y, Guo C, Li Y, Ma Y, Dong Z. Pharmacokinetic Interactions between Canagliflozin and Sorafenib or Lenvatinib in Rats. Molecules. 2022;27(17):5419. doi:10.3390/molecules27175419
22. Cui Y, Ma Y, Li Y, Song H, Dong Z. Influence of schisantherin A on the pharmacokinetics of lenvatinib in rats and its potential mechanism. J Gastrointest Oncol. 2022;13(2):802–811. doi:10.21037/jgo-22-174
23. Hata K, Suetsugu K, Egashira N, et al. Association of lenvatinib plasma concentration with clinical efficacy and adverse events in patients with hepatocellular carcinoma. Cancer Chemother Pharmacol. 2020;86(6):803–813. doi:10.1007/s00280-020-04178-x
24. Kumagai M, Nagahama M, Akamine Y, et al. Associations Between Plasma Concentrations of Lenvatinib and Angiopoietin and Clinical Responses to Lenvatinib Therapy in Japanese Patients With Thyroid Cancer. Cancer Diagn Progn. 2022;2(3):336–344. doi:10.21873/cdp.10114
25. Nagahama M, Ozeki T, Suzuki A, et al. Association of lenvatinib trough plasma concentrations with lenvatinib-induced toxicities in Japanese patients with thyroid cancer. Med Oncol. 2019;36(5):39. doi:10.1007/s12032-019-1263-3
26. Endo M, Honda K, Saito T, et al. Maximum Plasma Concentration of Lenvatinib Is Useful for Predicting Thrombocytopenia in Patients Treated for Hepatocellular Carcinoma. World J Oncol. 2021;12(5):165–172. doi:10.14740/wjon1399
27. Muraishi N, Kawamura Y, Akuta N, et al. The Impact of Lenvatinib on Tumor Blood Vessel Shrinkage of Hepatocellular Carcinoma during Treatment: an Imaging-Based Analysis. Oncology. 2023;101(2):134–144. doi:10.1159/000526976
28. Guo J, Zhao J, Xu Q, Huang D. Resistance of Lenvatinib in Hepatocellular Carcinoma. Curr Cancer Drug Targets. 2022;22(11):865–878. doi:10.2174/1568009622666220428111327
29. Tao M, Han J, Shi J, et al. Application and Resistance Mechanisms of Lenvatinib in Patients with Advanced Hepatocellular Carcinoma. J Hepatocell Carcinoma. 2023;10:1069–1083. doi:10.2147/JHC.S411806
30. Donne R, Lujambio A. The liver cancer immune microenvironment: therapeutic implications for hepatocellular carcinoma. Hepatology. 2023;77(5):1773–1796. doi:10.1002/hep.32740
31. Tang W, Chen Z, Zhang W, et al. The mechanisms of sorafenib resistance in hepatocellular carcinoma: theoretical basis and therapeutic aspects. Signal Transduct Target Ther. 2020;5(1):87. doi:10.1038/s41392-020-0187-x
32. Llovet JM, Ricci S, Mazzaferro V, et al. Sorafenib in advanced hepatocellular carcinoma. N Engl J Med. 2008;359(4):378–390. doi:10.1056/NEJMoa0708857
33. Li J, Zhu L, Kwok HF. Nanotechnology-based approaches overcome lung cancer drug resistance through diagnosis and treatment. Drug Resist Updat. 2023;66:100904. doi:10.1016/j.drup.2022.100904
34. Rosenzweig SA. Acquired Resistance to Drugs Targeting Tyrosine Kinases. Adv Cancer Res. 2018;138:71–98.
35. Motzer RJ, Taylor MH, Evans TRJ, et al. Lenvatinib dose, efficacy, and safety in the treatment of multiple malignancies. Expert Rev Anticancer Ther. 2022;22(4):383–400. doi:10.1080/14737140.2022.2039123
36. Zhou J, Ma L, Li Z, Chen B, Wu Y, Meng X. Synthesis of lenvatinib-loaded upconversion@polydopamine nanocomposites for upconversion luminescence imaging-guided chemo-photothermal synergistic therapy of anaplastic thyroid cancer. RSC Adv. 2023;13(38):26925–26932. doi:10.1039/D3RA02121A
37. Zhang S, Zhao L, Peng X, et al. Self-assembled phospholipid-based mixed micelles for improving the solubility, bioavailability and anticancer activity of lenvatinib. Colloids Surf B Biointerfaces. 2021;201:111644. doi:10.1016/j.colsurfb.2021.111644
38. Ning Z, Zhao Y, Yan X, Hua Y, Meng Z. Flower-like Composite Material Delivery of Co-Packaged Lenvatinib and Bufalin Prevents the Migration and Invasion of Cholangiocarcinoma. Nanomaterials (Basel). 2022;12(12):2048. doi:10.3390/nano12122048
39. Ning Z, Yang L, Yan X, et al. Effect and Mechanism of the Lenvatinib@H-MnO(2)-FA Drug Delivery System in Targeting Intrahepatic Cholangiocarcinoma. Curr Pharm Des. 2022;28(9):743–750. doi:10.2174/1381612828666220113161712
40. Zhang D, Jiang C, Zheng X, et al. Normalization of Tumor Vessels by Lenvatinib-Based Metallo-Nanodrugs Alleviates Hypoxia and Enhances Calreticulin-Mediated Immune Responses in Orthotopic HCC and Organoids. Small. 2023;19(29):e2207786. doi:10.1002/smll.202207786
41. Su M, Pang Z, Li L, et al. Charge-assisted bond and molecular self-assembly drive the gelation of lenvatinib mesylate. Int J Pharm. 2021;607:121019. doi:10.1016/j.ijpharm.2021.121019
42. Xu Q, Hu H, Mo Z, Chen T, He Q, Xu Z. A multifunctional nanotheranostic agent based on Lenvatinib for multimodal synergistic hepatocellular carcinoma therapy with remarkably enhanced efficacy. J Colloid Interface Sci. 2023;638:375–391. doi:10.1016/j.jcis.2023.01.144
43. Wu Y, Zhu R, Zhou M, et al. Homologous cancer cell membrane-camouflaged nanoparticles target drug delivery and enhance the chemotherapy efficacy of hepatocellular carcinoma. Cancer Lett. 2023;558:216106. doi:10.1016/j.canlet.2023.216106
44. Yoshida T, Kaibori M, Fujisawa N, et al. Efficacy of Nanofiber Sheets Incorporating Lenvatinib in a Hepatocellular Carcinoma Xenograft Model. Nanomaterials (Basel). 2022;12(8):1364. doi:10.3390/nano12081364
45. Liu X, Huangfu Y, Wang J, et al. Supramolecular Polymer-Nanomedicine Hydrogel Loaded with Tumor Associated Macrophage-Reprogramming polyTLR7/8a Nanoregulator for Enhanced Anti-Angiogenesis Therapy of Orthotopic Hepatocellular Carcinoma. Adv Sci (Weinh). 2023;10(22):e2300637. doi:10.1002/advs.202300637
46. Liu J, Chen J, Liu H, et al. Bi/Se-Based Nanotherapeutics Sensitize CT Image-Guided Stereotactic Body Radiotherapy through Reprogramming the Microenvironment of Hepatocellular Carcinoma. ACS Appl Mater Interfaces. 2021;13(36):42473–42485. doi:10.1021/acsami.1c11763
47. Ding L, Zhang P, Huang X, Yang K, Liu X, Yu Z. Intracellular Reduction-Responsive Molecular Targeted Nanomedicine for Hepatocellular Carcinoma Therapy. Front Pharmacol. 2021;12:809125. doi:10.3389/fphar.2021.809125
48. Giammona G, Drago SE, Calabrese G, et al. Galactosylated Polymer/Gold Nanorods Nanocomposites for Sustained and Pulsed Chemo-Photothermal Treatments of Hepatocarcinoma. Pharmaceutics. 2022;14(11):2503. doi:10.3390/pharmaceutics14112503
49. Xu Q, Li Q, Yang Z, et al. Lenvatinib and Cu(2-x)S nanocrystals co-encapsulated in poly(D,L-lactide-co-glycolide) for synergistic chemo-photothermal therapy against advanced hepatocellular carcinoma. J Mater Chem B. 2021;9(48):9908–9922. doi:10.1039/D1TB01808F
50. Luo Y, Wang J, Xu L, et al. A theranostic metallodrug modulates immunovascular crosstalk to combat immunosuppressive liver cancer. Acta Biomater. 2022;154:478–496. doi:10.1016/j.actbio.2022.10.032
51. Zheng C, Zhang W, Wang J, et al. Lenvatinib- and vadimezan-loaded synthetic high-density lipoprotein for combinational immunochemotherapy of metastatic triple-negative breast cancer. Acta Pharm Sin B. 2022;12(9):3726–3738. doi:10.1016/j.apsb.2022.02.021
52. Pan Y, Liu L, He Y, et al. NIR diagnostic imaging of triple-negative breast cancer and its lymph node metastasis for high-efficiency hypoxia-activated multimodal therapy. J Nanobiotechnology. 2023;21(1):312. doi:10.1186/s12951-023-02010-1
53. Skubalova Z, Rex S, Sukupova M, et al. Passive Diffusion vs Active pH-Dependent Encapsulation of Tyrosine Kinase Inhibitors Vandetanib and Lenvatinib into Folate-Targeted Ferritin Delivery System. Int J Nanomed. 2021;16:1–14. doi:10.2147/IJN.S275808
54. Wei G, Huang L, Jiang Y, et al. Lenvatinib-zinc phthalocyanine conjugates as potential agents for enhancing synergistic therapy of multidrug-resistant cancer by glutathione depletion. Eur J Med Chem. 2019;169:53–64. doi:10.1016/j.ejmech.2019.02.065
55. Zhou T, Hang D, Li Y, et al. Role of Gd(2)O(3)-doped carbon-11-choline-lenvatinib nanoparticles contrast agent PET/CT in the diagnosis of patients with lung cancer. Oncol Lett. 2020;19(2):1117–1124. doi:10.3892/ol.2019.11243
56. Yuan N, Zhang X, Cao Y, et al. Contrast-enhanced computerized tomography combined with a targeted nanoparticle contrast agent for screening for early-phase non-small cell lung cancer. Exp Ther Med. 2017;14(5):5063–5068. doi:10.3892/etm.2017.5140
57. Mehrdadi S. Drug Delivery of Solid Lipid Nanoparticles (SLNs) and Nanostructured Lipid Carriers (NLCs) to Target Brain Tumors. Adv Pharm Bull. 2023;13(3):512–520. doi:10.34172/apb.2023.062
58. Mo K, Kim A, Choe S, Shin M, Yoon H. Overview of Solid Lipid Nanoparticles in Breast Cancer Therapy. Pharmaceutics. 2023;15(8):2065. doi:10.3390/pharmaceutics15082065
59. Mondal A, Nayak AK, Chakraborty P, Banerjee S, Nandy BC. Natural Polymeric Nanobiocomposites for Anti-Cancer Drug Delivery Therapeutics: a Recent Update. Pharmaceutics. 2023;15(8):2064. doi:10.3390/pharmaceutics15082064
60. Pande S. Liposomes for drug delivery: review of vesicular composition, factors affecting drug release and drug loading in liposomes. Artif Cells Nanomed Biotechnol. 2023;51(1):428–440. doi:10.1080/21691401.2023.2247036
61. Tolba MM, Jabbar A, Afzal S, et al. A promising RNA nanotechnology in clinical therapeutics: a future perspective narrative review. Future Sci OA. 2023;9(8):FSO883. doi:10.2144/fsoa-2023-0067
62. Veerapandian M, Ramasundaram S, Jerome P, et al. Drug Delivery Application of Functional Nanomaterials Synthesized Using Natural Sources. J Funct Biomater. 2023;14(8):426. doi:10.3390/jfb14080426
63. Wen P, Ke W, Dirisala A, Toh K, Tanaka M, Li J. Stealth and pseudo-stealth nanocarriers. Adv Drug Deliv Rev. 2023;198:114895. doi:10.1016/j.addr.2023.114895
64. Gavas S, Quazi S, Karpinski TM. Nanoparticles for Cancer Therapy: current Progress and Challenges. Nanoscale Res Lett. 2021;16(1):173. doi:10.1186/s11671-021-03628-6
65. Bose R, Jayawant M, Raut R, et al. Cyclodextrin nanoparticles in targeted cancer theranostics. Front Pharmacol. 2023;14:1218867. doi:10.3389/fphar.2023.1218867
66. Ji W, Zhang Y, Deng Y, Li C, Kankala RK, Chen A. Nature-inspired nanocarriers for improving drug therapy of atherosclerosis. Regen Biomater. 2023;10:rbad069. doi:10.1093/rb/rbad069
67. Khan S, Sharma A, Jain V. An Overview of Nanostructured Lipid Carriers and its Application in Drug Delivery through Different Routes. Adv Pharm Bull. 2023;13(3):446–460. doi:10.34172/apb.2023.056
68. Koh HB, Kim HJ, Kang SW, Yoo TH. Exosome-Based Drug Delivery: translation from Bench to Clinic. Pharmaceutics. 2023;15(8):2042. doi:10.3390/pharmaceutics15082042
69. Korzun T, Moses AS, Diba P, et al. From Bench to Bedside: implications of Lipid Nanoparticle Carrier Reactogenicity for Advancing Nucleic Acid Therapeutics. Pharmaceuticals (Basel). 2023;16(8):1088. doi:10.3390/ph16081088
70. Doroudian M, MacLoughlin R, Poynton F, Prina-Mello A, Donnelly SC. Nanotechnology based therapeutics for lung disease. Thorax. 2019;74(10):965–976. doi:10.1136/thoraxjnl-2019-213037
71. Bayda S, Adeel M, Tuccinardi T, Cordani M, Rizzolio F. The History of Nanoscience and Nanotechnology: from Chemical-Physical Applications to Nanomedicine. Molecules. 2019;25(1):112. doi:10.3390/molecules25010112
72. Yuwen L, Zhang S, Chao J. Recent Advances in DNA Nanotechnology-Enabled Biosensors for Virus Detection. Biosensors. 2023;13(8). doi:10.3390/bios13080822
73. Li C, Zhang D, Pan Y, Chen B. Human Serum Albumin Based Nanodrug Delivery Systems: recent Advances and Future Perspective. Polymers (Basel). 2023;15(16).
74. Tang M, Chen B, Xia H, et al. pH-gated nanoparticles selectively regulate lysosomal function of tumour-associated macrophages for cancer immunotherapy. Nat Commun. 2023;14(1):5888. doi:10.1038/s41467-023-41592-0
75. Marshall SK, Angsantikul P, Pang Z, Nasongkla N, Hussen RSD, Thamphiwatana SD. Biomimetic Targeted Theranostic Nanoparticles for Breast Cancer Treatment. Molecules. 2022;27(19):6473. doi:10.3390/molecules27196473
76. Genchi GG, Marino A, Tapeinos C, Ciofani G. Smart Materials Meet Multifunctional Biomedical Devices: current and Prospective Implications for Nanomedicine. Front Bioeng Biotechnol. 2017;5:80. doi:10.3389/fbioe.2017.00080
77. Kong FH, Ye QF, Miao XY, et al. Current status of sorafenib nanoparticle delivery systems in the treatment of hepatocellular carcinoma. Theranostics. 2021;11(11):5464–5490. doi:10.7150/thno.54822
78. Ashique S, Garg A, Hussain A, Farid A, Kumar P, Taghizadeh-Hesary F. Nanodelivery systems: an efficient and target-specific approach for drug-resistant cancers. Cancer Med. 2023;12(18):18797–18825. doi:10.1002/cam4.6502
79. Pavelić K, Kraljević Pavelić S, Bulog A, et al. Nanoparticles in Medicine: current Status in Cancer Treatment. Int J Mol Sci. 2023;24(16):12827. doi:10.3390/ijms241612827
80. Jain RK. The next frontier of molecular medicine: delivery of therapeutics. Nature Med. 1998;4(6):655–657. doi:10.1038/nm0698-655
81. Fang J, Islam W, Maeda H. Exploiting the dynamics of the EPR effect and strategies to improve the therapeutic effects of nanomedicines by using EPR effect enhancers. Adv Drug Deliv Rev. 2020;157:142–160. doi:10.1016/j.addr.2020.06.005
82. Huang A, Yang XR, Chung WY, Dennison AR, Zhou J. Targeted therapy for hepatocellular carcinoma. Signal Transduct Target Ther. 2020;5(1):146.
83. Jain RK, Stylianopoulos T. Delivering nanomedicine to solid tumors. Nat Rev Clin Oncol. 2010;7(11):653–664. doi:10.1038/nrclinonc.2010.139
84. Olivier T, Galmarini CM, Patel K, Tannock IF. Drug resistance and the solid tumor microenvironment. J National Cancer Inst. 2007;99(19):1441–1454. doi:10.1093/jnci/djm135
85. Fu Y, Ye F, Zhang X, et al. Decrease in Tumor Interstitial Pressure for Enhanced Drug Intratumoral Delivery and Synergistic Tumor Therapy. ACS Nano. 2022;16(11):18376–18389. doi:10.1021/acsnano.2c06356
86. Wu C, Wang Z, Wang X, et al. Morphology/Interstitial Fluid Pressure-Tunable Nanopomegranate Designed by Alteration of Membrane Fluidity under Tumor Enzyme and PEGylation. Mol Pharm. 2021;18(5):2039–2052. doi:10.1021/acs.molpharmaceut.1c00036
87. Pérez-Herrero E, Lanier OL, Krishnan N, D’Andrea A, Peppas NA. Drug delivery methods for cancer immunotherapy. Drug Deliv Transl Res. 2023;14(1):30–61. doi:10.1007/s13346-023-01405-9
88. Wang Y, Zhang L, Zhao G, et al. Homologous targeting nanoparticles for enhanced PDT against osteosarcoma HOS cells and the related molecular mechanisms. J Nanobiotechnology. 2022;20(1):83. doi:10.1186/s12951-021-01201-y
89. Santos EDS, Nogueira KAB, Fernandes LCC, et al. EGFR targeting for cancer therapy: pharmacology and immunoconjugates with drugs and nanoparticles. Int J Pharm. 2021;592:120082. doi:10.1016/j.ijpharm.2020.120082
90. Mossenta M, Busato D, Dal Bo M, Macor P, Toffoli G. Novel Nanotechnology Approaches to Overcome Drug Resistance in the Treatment of Hepatocellular Carcinoma: glypican 3 as a Useful Target for Innovative Therapies. Int J Mol Sci. 2022;23(17):10038. doi:10.3390/ijms231710038
91. Mi X, Hu M, Dong M, et al. Folic Acid Decorated Zeolitic Imidazolate Framework (ZIF-8) Loaded with Baicalin as a Nano-Drug Delivery System for Breast Cancer Therapy. Int J Nanomed. 2021;16:8337–8352. doi:10.2147/IJN.S340764
92. Qi WW, Yu HY, Guo H, et al. Doxorubicin-loaded glycyrrhetinic acid modified recombinant human serum albumin nanoparticles for targeting liver tumor chemotherapy. Mol Pharm. 2015;12(3):675–683. doi:10.1021/mp500394v
93. Autio KA, Dreicer R, Anderson J, et al. Safety and Efficacy of BIND-014, a Docetaxel Nanoparticle Targeting Prostate-Specific Membrane Antigen for Patients With Metastatic Castration-Resistant Prostate Cancer: a Phase 2 Clinical Trial. JAMA Oncol. 2018;4(10):1344–1351. doi:10.1001/jamaoncol.2018.2168
94. Fabi A, Ferretti G, Malaguti P, et al. Nanoparticle albumin-bound paclitaxel/liposomal-encapsulated doxorubicin in HER2-negative metastatic breast cancer patients. Future Oncol. 2020;16(22):1629–1637. doi:10.2217/fon-2019-0742
95. Hossen S, Hossain MK, Basher MK, Mia MNH, Rahman MT, Uddin MJ. Smart nanocarrier-based drug delivery systems for cancer therapy and toxicity studies: a review. J Adv Res. 2019;15:1–18. doi:10.1016/j.jare.2018.06.005
96. Li J, Kataoka K. Chemo-physical Strategies to Advance the in Vivo Functionality of Targeted Nanomedicine: the Next Generation. J Am Chem Soc. 2021;143(2):538–559. doi:10.1021/jacs.0c09029
97. Caldorera-Moore M, Guimard N, Shi L, Roy K. Designer nanoparticles: incorporating size, shape and triggered release into nanoscale drug carriers. Expert Opin Drug Deliv. 2010;7(4):479–495. doi:10.1517/17425240903579971
98. Gao Y, Shi Y, Fu M, et al. Simulation study of the effects of interstitial fluid pressure and blood flow velocity on transvascular transport of nanoparticles in tumor microenvironment. Comput Methods Programs Biomed. 2020;193:105493. doi:10.1016/j.cmpb.2020.105493
99. Xiao Y, Yu D. Tumor microenvironment as a therapeutic target in cancer. Pharmacol Ther. 2021;221:107753. doi:10.1016/j.pharmthera.2020.107753
100. Su MC, Nethi SK, Dhanyamraju PK, Prabha S. Nanomedicine Strategies for Targeting Tumor Stroma. Cancers (Basel). 2023;15(16):4145. doi:10.3390/cancers15164145
101. Ribeiro TP, Moreira JA, Monteiro FJ, Laranjeira MS. Nanomaterials in cancer: reviewing the combination of hyperthermia and triggered chemotherapy. J Control Release. 2022;347:89–103. doi:10.1016/j.jconrel.2022.04.045
102. Homsirikamol C, Suvanasuthi S, Viravaidya-Pasuwat K. Inclusion of IR-820 into Soybean-Phosphatides-Based Nanoparticles for Near-Infrared-Triggered Release and Endolysosomal Escape in HaCaT Keratinocytes at Insignificant Cytotoxic Level. Int J Nanomed. 2020;15:8717–8737. doi:10.2147/IJN.S267119
103. Gan Z, Zhang T, Liu Y, Wu D. Temperature-triggered enzyme immobilization and release based on cross-linked gelatin nanoparticles. PLoS One. 2012;7(10):e47154. doi:10.1371/journal.pone.0047154
104. Hu T, Huang Y, Liu J, Shen C, Wu F, He Z. Biomimetic Cell-Derived Nanoparticles: emerging Platforms for Cancer Immunotherapy. Pharmaceutics. 2023;15(7):1821. doi:10.3390/pharmaceutics15071821
105. Jiang Q, Xie M, Chen R, et al. Cancer cell membrane-wrapped nanoparticles for cancer immunotherapy: a review of current developments. Front Immunol. 2022;13:973601. doi:10.3389/fimmu.2022.973601
106. Wang Y, Zhang K, Li T, et al. Macrophage membrane functionalized biomimetic nanoparticles for targeted anti-atherosclerosis applications. Theranostics. 2021;11(1):164–180. doi:10.7150/thno.47841
107. Zhao X, Yan C. Research Progress of Cell Membrane Biomimetic Nanoparticles for Tumor Therapy. Nanoscale Res Lett. 2022;17(1):36. doi:10.1186/s11671-022-03673-9
108. Huang ZL, Li F, Zhang JT, et al. Research on the Construction of Bispecific-Targeted Sustained-Release Drug-Delivery Microspheres and Their Function in Treatment of Hepatocellular Carcinoma. ACS Omega. 2022;7(25):22003–22014.
109. Yu Z, Guo J, Hu M, Gao Y, Huang L. Icaritin Exacerbates Mitophagy and Synergizes with Doxorubicin to Induce Immunogenic Cell Death in Hepatocellular Carcinoma. ACS Nano. 2020;14(4):4816–4828. doi:10.1021/acsnano.0c00708
110. Hewett Y, Ghimire S, Farooqi B, Shah BK. Lenvatinib - A multikinase inhibitor for radioiodine-refractory differentiated thyroid cancer. J Oncol Pharm Pract. 2018;24(1):28–32. doi:10.1177/1078155216680119
111. Ielasi L, Tovoli F, Piscaglia F. Lenvatinib mesylate to treat hepatocellular carcinoma. Drugs Today (Barc). 2019;55(5):305–313. doi:10.1358/dot.2019.55.5.2969817
112. Niiyama E, Uto K, Lee CM, Sakura K, Ebara M. Hyperthermia Nanofiber Platform Synergized by Sustained Release of Paclitaxel to Improve Antitumor Efficiency. Adv Healthc Mater. 2019;8(13):e1900102. doi:10.1002/adhm.201900102
113. Chen X, Zhang L, Wang X, et al. Stat3 shRNA delivery with folate receptor-modified multi-functionalized graphene oxide particles for combined infrared radiation and gene therapy in hepatocellular carcinoma. Anticancer Drugs. 2023;34(6):715–724. doi:10.1097/CAD.0000000000001461
114. Ngernyuang N, Seubwai W, Daduang S, Boonsiri P, Limpaiboon T, Daduang J. Targeted delivery of 5-fluorouracil to cholangiocarcinoma cells using folic acid as a targeting agent. Mater Sci Eng C Mater Biol Appl. 2016;60:411–415. doi:10.1016/j.msec.2015.11.062
115. Oura K, Morishita A, Hamaya S, Fujita K, Masaki T. The Roles of Epigenetic Regulation and the Tumor Microenvironment in the Mechanism of Resistance to Systemic Therapy in Hepatocellular Carcinoma. Int J Mol Sci. 2023;24(3).
116. Santhakumar C, Gane EJ, Liu K, McCaughan GW. Current perspectives on the tumor microenvironment in hepatocellular carcinoma. Hepatol Internat. 2020;14(6):947–957. doi:10.1007/s12072-020-10104-3
117. Satilmis B, Sahin TT, Cicek E, Akbulut S, Yilmaz S. Hepatocellular Carcinoma Tumor Microenvironment and Its Implications in Terms of Anti-tumor Immunity: future Perspectives for New Therapeutics. J Gastrointest Cancer. 2021;52(4):1198–1205. doi:10.1007/s12029-021-00725-8
118. Wu Q, Hu Y, Yu B, Hu H, Xu F-J. Polysaccharide-based tumor microenvironment-responsive drug delivery systems for cancer therapy. J Control Release. 2023;362:19–43. doi:10.1016/j.jconrel.2023.08.019
119. Chen C, Wang Z, Ding Y, Qin Y. Tumor microenvironment-mediated immune evasion in hepatocellular carcinoma. Front Immunol. 2023;14:1133308. doi:10.3389/fimmu.2023.1133308
120. Kopecka J, Salaroglio IC, Perez-Ruiz E, et al. Hypoxia as a driver of resistance to immunotherapy. Drug Resist Updates. 2021;59.
121. Xu B, Shaoyong W, Wang L, et al. Gut-targeted nanoparticles deliver specifically targeted antimicrobial peptides against Clostridium perfringens infections. Sci Adv. 2023;9(39):eadf8782. doi:10.1126/sciadv.adf8782
122. Kalluri R. The biology and function of fibroblasts in cancer. Nat Rev Cancer. 2016;16(9):582–598. doi:10.1038/nrc.2016.73
123. Lu Y, Feng N, Du Y, Yu R. Nanoparticle-Based Therapeutics to Overcome Obstacles in the Tumor Microenvironment of Hepatocellular Carcinoma. Nanomaterials (Basel). 2022;12(16):2832. doi:10.3390/nano12162832
124. Dong S, Dong Y, Jia T, et al. GSH-Depleted Nanozymes with Hyperthermia-Enhanced Dual Enzyme-Mimic Activities for Tumor Nanocatalytic Therapy. Adv Mater. 2020;32(42):e2002439. doi:10.1002/adma.202002439
125. Feng X, Xu W, Liu J, et al. Polypeptide nanoformulation-induced immunogenic cell death and remission of immunosuppression for enhanced chemoimmunotherapy. Sci Bull. 2021;66(4):362–373. doi:10.1016/j.scib.2020.07.013
126. Huang K, Shi B, Xu W, et al. Reduction-responsive polypeptide nanogel delivers antitumor drug for improved efficacy and safety. Acta Biomater. 2015;27:179–193. doi:10.1016/j.actbio.2015.08.049
127. Monteiro PF, Travanut A, Conte C, Alexander C. Reduction-responsive polymers for drug delivery in cancer therapy-Is there anything new to discover? Wiley Interdiscip Rev Nanomed Nanobiotechnol. 2021;13(2):e1678. doi:10.1002/wnan.1678
128. Jiang B, Fang L, Wu K, Yan X, Fan K. Ferritins as natural and artificial nanozymes for theranostics. Theranostics. 2020;10(2):687–706. doi:10.7150/thno.39827
129. Li Y, Gao H, Nepovimova E, Wu Q, Adam V, Kuca K. Recombinant ferritins for multimodal nanomedicine. J Enzyme Inhib Med Chem. 2023;38(1):2219868. doi:10.1080/14756366.2023.2219868
130. Chen Y, Zeng L, Zhu H, et al. Ferritin Nanocaged Doxorubicin Potentiates Chemo-Immunotherapy against Hepatocellular Carcinoma via Immunogenic Cell Death. Small Methods. 2023;7(5):e2201086. doi:10.1002/smtd.202201086
131. Farooq U, Wang H, Hu J, et al. Polydatin Inhibits Hepatocellular Carcinoma Cell Proliferation and Sensitizes Doxorubicin and Cisplatin through Targeting Cell Mitotic Machinery. Cells. 2023;12(2):222. doi:10.3390/cells12020222
132. Kciuk M, Gielecińska A, Mujwar S, et al. Doxorubicin-An Agent with Multiple Mechanisms of Anticancer Activity. Cells. 2023;12(4).
133. Hu S, Dan W, Liu J, et al. The Use of Traditional Chinese Medicine in Relieving EGFR-TKI-Associated Diarrhea Based on Network Pharmacology and Data Mining. Evid Based Complement Alternat Med. 2021;2021:5530898. doi:10.1155/2021/5530898
134. Chen Q, Shu C, Laurence AD, et al. Effect of Huaier granule on recurrence after curative resection of HCC: a multicentre, randomised clinical trial. Gut. 2018;67(11):2006–2016. doi:10.1136/gutjnl-2018-315983
135. Qi J, Xie FJ, Liu S, et al. Huaier Granule Combined with Tegafur Gimeracil Oteracil Potassium Promotes Stage IIb Gastric Cancer Prognosis and Induces Gastric Cancer Cell Apoptosis by Regulating Livin. Biomed Res Int. 2020;2020:2403595. doi:10.1155/2020/2403595
136. Jiang HY, Zheng HM, Xia C, et al. The Research Progress of Bufalin in the Treatment of Hepatocellular Carcinoma. Onco Targets Ther. 2022;15:291–298. doi:10.2147/OTT.S333233
137. Soumoy L, Ghanem GE, Saussez S, Journe F. Bufalin for an innovative therapeutic approach against cancer. Pharmacol Res. 2022;184:106442. doi:10.1016/j.phrs.2022.106442
138. Yu Z, Li Y, Li Y, et al. Bufalin stimulates antitumor immune response by driving tumor-infiltrating macrophage toward M1 phenotype in hepatocellular carcinoma. J Immunother Cancer. 2022;10(5):e004297. doi:10.1136/jitc-2021-004297
139. Wang H, Zhang C, Chi H, Meng Z. Synergistic anti-hepatoma effect of bufalin combined with sorafenib via mediating the tumor vascular microenvironment by targeting mTOR/VEGF signaling. Int J Oncol. 2018;52(6):2051–2060. doi:10.3892/ijo.2018.4351
140. Chen LC, Lin HY, Hung SK, Chiou WY, Lee MS. Role of modern radiotherapy in managing patients with hepatocellular carcinoma. World J Gastroenterol. 2021;27(20):2434–2457. doi:10.3748/wjg.v27.i20.2434
141. Yoon SM, Ryoo BY, Lee SJ, et al. Efficacy and Safety of Transarterial Chemoembolization Plus External Beam Radiotherapy vs Sorafenib in Hepatocellular Carcinoma With Macroscopic Vascular Invasion: a Randomized Clinical Trial. JAMA Oncol. 2018;4(5):661–669. doi:10.1001/jamaoncol.2017.5847
142. Rim CH, Lee HY, Kim JS, Kim H. Radiofrequency ablation and stereotactic body radiotherapy for hepatocellular carcinoma: should they clash or reconcile? Int J Radiat Biol. 2021;97(2):111–119. doi:10.1080/09553002.2021.1857453
143. Jarosz-Biej M, Smolarczyk R, Cichoń T, Kułach N. Tumor Microenvironment as A Game Changer in Cancer Radiotherapy. Int J Mol Sci. 2019;20(13):3212. doi:10.3390/ijms20133212
144. Payne M, Bossmann SH, Basel MT. Direct treatment versus indirect: thermo-ablative and mild hyperthermia effects. Wiley Interdiscip Rev Nanomed Nanobiotechnol. 2020;12(5):e1638. doi:10.1002/wnan.1638
145. Brace C. Thermal tumor ablation in clinical use. IEEE Pulse. 2011;2(5):28–38. doi:10.1109/MPUL.2011.942603
146. Lee S, Son B, Park G, et al. Immunogenic Effect of Hyperthermia on Enhancing Radiotherapeutic Efficacy. Int J Mol Sci. 2018;19(9).
147. Balivada S, Rachakatla RS, Wang H, et al. A/C magnetic hyperthermia of melanoma mediated by iron(0)/iron oxide core/shell magnetic nanoparticles: a mouse study. BMC Cancer. 2010;10(1):119. doi:10.1186/1471-2407-10-119
148. Melamed JR, Edelstein RS, Day ES. Elucidating the fundamental mechanisms of cell death triggered by photothermal therapy. ACS Nano. 2015;9(1):6–11. doi:10.1021/acsnano.5b00021
149. Kokalari I, Keshavan S, Rahman M, et al. Efficacy, biocompatibility and degradability of carbon nanoparticles for photothermal therapy of lung cancer. Nanomedicine (Lond). 2021;16(9):689–707. doi:10.2217/nnm-2021-0009
150. Alamdari SG, Amini M, Jalilzadeh N, et al. Recent advances in nanoparticle-based photothermal therapy for breast cancer. J Control Release. 2022;349:269–303. doi:10.1016/j.jconrel.2022.06.050
151. Kong C, Chen X. Combined Photodynamic and Photothermal Therapy and Immunotherapy for Cancer Treatment: a Review. Int J Nanomed. 2022;17:6427–6446. doi:10.2147/IJN.S388996
152. Dariva CG, Coelho JFJ, Serra AC. Near infrared light-triggered nanoparticles using singlet oxygen photocleavage for drug delivery systems. J Control Release. 2019;294:337–354. doi:10.1016/j.jconrel.2018.12.042
153. Xu Y, Kim CS, Saylor DM, Koo D. Polymer degradation and drug delivery in PLGA-based drug-polymer applications: a review of experiments and theories. J Biomed Mater Res B Appl Biomater. 2017;105(6):1692–1716. doi:10.1002/jbm.b.33648
154. Kadkhoda J, Tarighatnia A, Barar J, Aghanejad A, Davaran S. Recent advances and trends in nanoparticles based photothermal and photodynamic therapy. Photodiagnosis Photodyn Ther. 2022;37:102697. doi:10.1016/j.pdpdt.2021.102697
155. Ji B, Wei M, Yang B. Recent advances in nanomedicines for photodynamic therapy (PDT)-driven cancer immunotherapy. Theranostics. 2022;12(1):434–458. doi:10.7150/thno.67300
156. Hou YJ, Yang XX, Liu RQ, et al. Pathological Mechanism of Photodynamic Therapy and Photothermal Therapy Based on Nanoparticles. Int J Nanomed. 2020;15:6827–6838. doi:10.2147/IJN.S269321
157. Zong J, Peng H, Qing X, et al. pH-Responsive Pluronic F127-Lenvatinib-Encapsulated Halogenated Boron-Dipyrromethene Nanoparticles for Combined Photodynamic Therapy and Chemotherapy of Liver Cancer. ACS Omega. 2021;6(18):12331–12342. doi:10.1021/acsomega.1c01346
158. Wang Y, Tang Y, Du Y, et al. Genetically engineered bacteria-mediated multi-functional nanoparticles for synergistic tumor-targeting therapy. Acta Biomater. 2022;150:337–352. doi:10.1016/j.actbio.2022.07.056
159. Song F, Li S, Sun C, Ji Y, Zhang Y. ROS-Responsive Selenium-Containing Carriers for Coencapsulation of Photosensitizer and Hypoxia-Activated Prodrug and Their Cellular Behaviors. Macromol Biosci. 2021;21(12):e2100229. doi:10.1002/mabi.202100229
160. Zhang W, Li J, Chen L, Chen H, Zhang L. Palladium-based multifunctional nanoparticles for combined chemodynamic/photothermal and calcium overload therapy of tumors. Colloids Surf B Biointerfaces. 2023;230:113529. doi:10.1016/j.colsurfb.2023.113529
161. Lu H, Xu S, Ge G, Guo Z, Zhao M, Liu Z. Boosting Chemodynamic Therapy by Tumor-Targeting and Cellular Redox Homeostasis-Disrupting Nanoparticles. ACS Appl Mater Interfaces. 2022;14(39):44098–44110. doi:10.1021/acsami.2c11091
162. Wang Y, Wang D, Zhang Y, et al. Tumor Microenvironment-Adaptive Nanoplatform Synergistically Enhances Cascaded Chemodynamic Therapy. Bioact Mater. 2023;22:239–253. doi:10.1016/j.bioactmat.2022.09.025
163. Sies H, Jones DP. Reactive oxygen species (ROS) as pleiotropic physiological signalling agents. Nat Rev Mol Cell Biol. 2020;21(7):363–383. doi:10.1038/s41580-020-0230-3
164. Jia C, Guo Y, Wu FG. Chemodynamic Therapy via Fenton and Fenton-Like Nanomaterials: strategies and Recent Advances. Small. 2022;18(6):e2103868. doi:10.1002/smll.202103868
165. Eggert T, Greten TF. Tumor regulation of the tissue environment in the liver. Pharmacol Ther. 2017;173:47–57. doi:10.1016/j.pharmthera.2017.02.005
166. Jayson GC, Kerbel R, Ellis LM, Harris AL. Antiangiogenic therapy in oncology: current status and future directions. Lancet. 2016;388(10043):518–529. doi:10.1016/S0140-6736(15)01088-0
167. Cassetta L, Pollard JW. Targeting macrophages: therapeutic approaches in cancer. Nat Rev Drug Discov. 2018;17(12):887–904. doi:10.1038/nrd.2018.169
168. Geeva NS. Lithium entrapped chitosan nanoparticles to reduce toxicity and increase cellular uptake of lithium. Environ Toxicol Pharmacol. 2018;61:79–86. doi:10.1016/j.etap.2018.05.017
169. Kefeni KK, Msagati TAM, Nkambule TT, Mamba BB. Spinel ferrite nanoparticles and nanocomposites for biomedical applications and their toxicity. Mater Sci Eng C Mater Biol Appl. 2020;107:110314. doi:10.1016/j.msec.2019.110314
170. Lu W, Yao J, Zhu X, Qi Y. Nanomedicines: redefining traditional medicine. Biomed Pharmacother. 2021;134:111103. doi:10.1016/j.biopha.2020.111103
171. Elnaggar MH, Abushouk AI, Hassan AHE, et al. Nanomedicine as a putative approach for active targeting of hepatocellular carcinoma. Semi Cancer Biol. 2021;69:91–99. doi:10.1016/j.semcancer.2019.08.016
172. Umer A, Ghouri MD, Muyizere T, et al. Engineered Nano-Bio Interfaces for Stem Cell Therapy. Precis Chem. 2023;1(6):341–356. doi:10.1021/prechem.3c00056
173. Scaranti M, Cojocaru E, Banerjee S, Banerji U. Exploiting the folate receptor α in oncology. Nat Rev Clin Oncol. 2020;17(6):349–359. doi:10.1038/s41571-020-0339-5
174. Chidambaranathan-Reghupaty S, Fisher PB, Sarkar D. Hepatocellular carcinoma (HCC): epidemiology, etiology and molecular classification. Adv Cancer Res. 2021;149:1–61.
175. Ho DW, Tsui YM, Chan LK, et al. Single-cell RNA sequencing shows the immunosuppressive landscape and tumor heterogeneity of HBV-associated hepatocellular carcinoma. Nat Commun. 2021;12(1):3684. doi:10.1038/s41467-021-24010-1
176. Milewska S, Niemirowicz-Laskowska K, Siemiaszko G, Nowicki P, Wilczewska AZ, Car H. Current Trends and Challenges in Pharmacoeconomic Aspects of Nanocarriers as Drug Delivery Systems for Cancer Treatment. Int J Nanomed. 2021;16:6593–6644. doi:10.2147/IJN.S323831
177. Saifi MA, Khan W, Godugu C. Cytotoxicity of Nanomaterials: using Nanotoxicology to Address the Safety Concerns of Nanoparticles. Pharm Nanotechnol. 2018;6(1):3–16. doi:10.2174/2211738505666171023152928
178. Kawasaki R, Tsuchiya K, Kodama Y, Numata K. Development of Reactive Oxygen Species-Triggered Degradable Nanoparticles Using Oligoproline-Containing Peptides. Biomacromolecules. 2020;21(10):4116–4122. doi:10.1021/acs.biomac.0c00915
179. Qi Y, Xu H, Li X, et al. Silica nanoparticles induce cardiac injury and dysfunction via ROS/Ca(2+)/CaMKII signaling. Sci Total Environ. 2022;837:155733. doi:10.1016/j.scitotenv.2022.155733
180. Zhang Y, Wu X, Zhu H, Cong Y. Development and in functional study of a bi-specific sustained release drug-loaded nano-liposomes for hepatocellular carcinoma. J Biomater Appl. 2023;38(1):97–108. doi:10.1177/08853282231179313
181. Yang W, Lee JC, Chen MH, et al. Thermosensitive liposomal doxorubicin plus radiofrequency ablation increased tumor destruction and improved survival in patients with medium and large hepatocellular carcinoma: a randomized, double-blinded, dummy-controlled clinical trial in a single center. J Cancer Res Ther. 2019;15(4):773–783. doi:10.4103/jcrt.JCRT_801_18
182. Su D. The transcatheter arterial chemoembolization combined with targeted nanoparticle delivering sorafenib system for the treatment of microvascular invasion of hepatocellular carcinoma. Bioengineered. 2021;12(2):11124–11135. doi:10.1080/21655979.2021.2001239
© 2024 The Author(s). This work is published and licensed by Dove Medical Press Limited. The
full terms of this license are available at https://www.dovepress.com/terms.php
and incorporate the Creative Commons Attribution
- Non Commercial (unported, v3.0) License.
By accessing the work you hereby accept the Terms. Non-commercial uses of the work are permitted
without any further permission from Dove Medical Press Limited, provided the work is properly
attributed. For permission for commercial use of this work, please see paragraphs 4.2 and 5 of our Terms.